Chapter 50C Trauma of the Nervous System
Spinal Cord Trauma
Transient Spinal Cord Syndromes
Mechanisms and Types of Injuries
Management of Acute Spinal Cord Injuries
Treatment of Spinal Cord Injuries in the Acute Setting
Long-Term Management of Spinal Cord Injuries
Delayed Posttraumatic Spinal Cord Syndromes
Sexual Dysfunction, Sexuality, and Fertility in Spinal Cord Injuries
Deep Vein Thrombosis and Thromboembolism in Spinal Cord Injury
Epidemiology
The annual incidence of SCI worldwide is between 11.5 and 57.8 cases per million persons (Ackery et al., 2004). In the United States, the annual incidence is approximately 40 cases per million, with approximately 12,000 new cases diagnosed a year. There is a bimodal age distribution, with the highest frequency occurring between 15 and 29 years of age and the second occurring at 65 years of age and older (van den Berg et al., 2010b). On a global scale, inconsistencies in reporting and failure to include patients who died before entering a hospital lead to wide discrepancies in the actual incidence. The mortality rate associated with SCIs between the time of the event and the time of presentation to the hospital is between 48.3% and 79% (Kraus et al., 1975). Once admitted to a hospital, the mortality rate decreases and is reported to be between 4.4% and 16.7% (Kraus, 1980). The leading cause of death in patients with SCI relates to respiratory complications (van den Berg et al., 2010). In North American trauma centers, approximately 1 in 40 patients admitted suffers from an acute SCI (Burney et al., 1993). The present estimation of SCI victims is reported to be 259,000 (Spinal Cord Injury Information Network, 2009). The two most common causes of SCIs are motor vehicle collisions and falls (van den Berg et al., 2010). Other causes of SCI include work-related injuries, sports and recreational injuries, and violence. Spinal cord injuries predominantly occur in the younger population (20-40 years of age); age 35 is the worldwide average, and age 28.7 is the U.S. average. Typically, SCIs occur more commonly in males than females by a factor of 3 or 4 (Sekhon and Fehlings, 2001).
Pathophysiology
Secondary injuries are divided into acute, intermediate, and chronic stages. The acute phase is divided into an early acute phase and a subacute phase. The biochemical processes occurring in the early acute phase of injury are targeted for neuroprotective therapies. Ionic homeostasis is desynchronized during this period and contributes to apoptosis and necrotic cell death. In particular, Ca2+ deregulation leads to a variety of damaging processes such as mitochondrial dysfunction. This in turn leads to low adenosine triphosphate (ATP) levels. Without enough ATP to sustain energy-dependent transporters such as the Na+/K+-ATPase membrane transporter, ionic homeostasis is further disrupted. This disruption of ionic homeostasis leads to failure of the Na+/K+/glutamate pump, which conceivably leads to elevated levels of glutamate. Glutamate in turn acts on a variety of glutamate receptors such as N-methyl-d-aspartate (NMDA), alpha-amino-3-hydroxy-5-methyl-4-isoxazolepropionic acid (AMPA), and kainite receptors, leading to an influx of Na+ and Ca2+. Free radical reactions create membrane damage via lipid peroxidation, further promoting cell lysis, dysfunction of organelles, and calcium deregulation. Free radical production peaks at 12 hours post injury and continues to have an active presence for another week before returning to preinjury levels at 4 to 5 weeks. Recently it has been found that the primary mediator of free radical injury is the peroxynitrite radical (Xiong et al., 2007). In rats, the peroxynitrite radical has been shown to cause apoptosis (Bao and Liu, 2003). Antioxidants and inhibitors of peroxynitrite radicals have shown promise as neuroprotective elements. One such compound, methylprednisolone, is commonly used because of its suspected role in the inhibition of lipid peroxidation.
Following injury to the spinal cord, the blood-brain barrier has a higher permeability due to injured endothelial cells and astrocytic processes and inflammatory mediators that increase vascular permeability. Animal studies show the peak vascular permeability occurring at 24 hours and tapering off over a 2-week period (Noble and Wrathall, 1989). In humans, the time course is suspected to be the same. Two mediators upregulated to increase vascular permeability are TNF-α and IL-1β. Other compounds found to have negative effects on the permeability of the blood-brain barrier include reactive oxygen species (ROS; e.g., nitric oxide), histamine, matrix metalloproteineases, and elastase.
Despite the inflammatory response exerting deleterious effects, it is crucial in maintaining an environment for regenerative growth and removing cellular debris. Animal studies have been used to study the changes that occur on the cellular level. Recently, spinal cords taken from autopsy specimens of patients suffering from SCIs were used to study the changes occurring at the cellular level (Fleming et al., 2006). This study showed the presence of neutrophils at the injured sites within 4 hours post injury. After peaking between 1 and 3 days post injury, they remained present for as long as 10 days. Microglial cells were also shown to be an important component of the early inflammatory process. They became activated and increased in number during the first 3 days post injury. Like the neutrophils, their presence correlated with areas of increased tissue damage. During the following 5- to 10-day period post injury, the predominant cell population transitioned to the activated microglia and macrophages. Macrophages with a phagocytic phenotype were noted to be CD68 reactive (Schmitt et al., 2000). Over months to years after injury, most of the foamy macrophages seen were no longer CD68 reactive (Fleming et al., 2006). This is thought to be due to decreased phagocytic activity of existing macrophages, leading to reduced expression of the lysosomal protein. Currently it is felt that macrophages have a lifespan of 4 weeks but do not express a phagocytic phenotype for this entire duration (Ross and Auger, 2002). Furthermore, macrophages that arrive in chronic lesions may not be influenced by the local environment to produce CD68. Recently it has been felt that the secretion of oxidative and proteolytic enzymes by neutrophils, activated microglia, and macrophages during the first 3 days post injury imparts a high degree of secondary injury to the spinal cord. Noncellular mediators that contribute to this process include TNF-α, interferons, and interleukins, as discussed. Inhibition of TNF-α has been found to promote recovery following SCI (Bethea et al., 1999). However, TNF-α has been found to be neuroprotective in vitro (Cheng et al., 1994) and in studies with TNF-α–deficient mice (Kim et al., 2001). Thus, the exact role of TNF-α in SCI must be better defined before future therapeutic modalities can capitalize on the manipulation of this mediator.
Cell death following SCI occurs by one of two mechanisms: apoptosis or necrosis. Potentially, a newly discovered mechanism of cell death known as necroptosis can cause a programmed necrotic event to occur (Galluzzi and Kroemer, 2008). Apoptosis has not been well documented in human SCIs, but there is a substantial amount of literature on the topic in animal SCIs. Following SCI, there is expression of Fas ligand by microglia and lymphocytes and FasR by oligodendrocytes (Casha et al., 2001, 2005; Nagata et al., 1995). It is well acknowledged that one potential method of initiating the caspase cascade is through the interaction of the Fas ligand and Fas receptor. Proteolysis and deoxyribonucleic acid (DNA) cleavage are part of apoptosis. Blocking the caspase cascade blocks apoptosis. In animal studies, apoptosis readily occurs in oligodendrocytes following ischemic injury, thus resulting in axonal demyelination (Totiu et al., 2005). This phenomenon is not clearly witnessed in postmortem examination of human SCI (Kakulas, 2004; Norenberg et al., 2004).
The subacute period lasts from 2 days to 2 weeks. It is during this time that the phagocytic response is responsible for removing cellular debris. The removal of growth-inhibiting compounds found in myelin debris can potentially have some beneficial effects on the efforts of axonal recovery (Donnelly and Popovich, 2007). Astrocytes also reach peak numbers in the subacute period. They form a scar that prevents axonal regeneration in rodent studies. The presence of the astroglial scar is less obvious in humans (Hagg and Oudega, 2006). Despite suspected negative effects on healing, they have important roles in ionic homeostasis and reestablishing the blood-brain barrier, thus limiting the immigration of immune cells and edema.
Clinical Presentation
The majority of SCIs occur in the cervical spine (55%) (Sekhon and Fehlings, 2001). Other injuries are evenly divided among the thoracic, thoracolumbar, and lumbar regions. The most frequent injuries suffered are incomplete tetraplegia followed by complete paraplegia, complete tetraplegia, and incomplete paraplegia.
In general, SCIs can be categorized into complete injuries and incomplete injuries. In complete injuries, there is an absence of motor, sensory, and bowel and bladder function below the level of injury. There is some preservation of neurological function with incomplete injuries. At present, SCIs are graded using the American Spinal Injury Association/International Medical Society of Paraplegia (ASIA/IMSOP) Impairment Scale (Box 50C.1) in conjunction with motor grading provided by the Medical Research Council Muscle Grading System (Table 50C.1). This grading system provides a standard method by which clinicians and researchers can classify SCIs. In defining the level of the injury, the most caudal segment at which there is normal motor and sensory function is taken into account. This may differ from the level in the vertebral column where the injury occurred.
Box 50C.1
ASIA/IMSOP Impairment Scale
Modified and reprinted with permission from the American Spinal Injury Society (ASIA) and International Medical Society of Paraplegia (IMSOP).
Table 50C.1 Medical Research Council Muscle Grading System
Grade | Physical Examination Finding |
---|---|
5 | Full ROM against full resistance |
4+ | Full ROM against nearly full resistance |
4 | Full ROM against moderate resistance |
4− | Full ROM against some resistance |
3 | Full ROM against gravity |
2 | Full ROM with gravity eliminated |
1 | Partial or trace muscle contraction |
0 | No muscular contraction |
ROM, Range of motion.
Modified from Aids to the Examination of the Peripheral Nervous System, 1986. Baillière Tindall on behalf of the Guarantors of Brain, London.
Spinal Cord Injury Syndromes
Central Cord Syndrome
Central cord syndrome is present in 9% of all traumatic cord injuries and is the most common of the spinal cord syndromes. This is a condition first reported by Thornburn in 1887 and then popularized by Schneider et al. in 1954. Hyperextension in the cervical spine, with some preexisting cervical spondylosis, is usually responsible for this type of injury. Imaging the cervical spine in patients with central cord syndrome will reveal stenosis from spondylosis, fracture subluxation, or sequestered disk, with no spinal stenosis. Schneider proposed that these injuries resulted from acute compression from preexisting bone spurs anteriorly and hypertrophied ligamentum flavum posteriorly, and contributed to hematomyelia and central cord necrosis (Fig. 50C.1). Schneider witnessed weakness in the upper extremities greater than the lower extremities, as well as a variable degree of sensory disturbances and loss of bladder control. It was proposed that pyramidal signs due to involvement of the anterior horn cells led to weakness in the arms greater than the legs, secondary to the topography of the corticospinal tracts. Because of their good recovery, Schneider was in favor of taking a more conservative approach toward treating these patients. In his case series, he did note that the majority of his patients had permanent disability in their hands that rarely recovered. An alternative explanation takes into account the belief that a greater focus of the corticospinal tracts is dedicated to supplying the distal musculature in the upper extremity (Levi et al., 1996). Thus, injury to the corticospinal tract produces more significant weakness in the upper extremity, with concentration in the hands. Correlations of magnetic resonance imaging (MRI) (Quencer et al., 1992) and histopathology (Martin et al., 1992; Jimenez et al., 2000) fail to suggest hematomyelia from Schneider’s hypothesis. There is in fact minimal disruption of the central gray matter. Axonal disruption and swelling is more widespread in the white matter.
Anterior Cord Syndrome
Anterior cord syndrome occurs with injuries to the ventral two-thirds of the cord, while sparing the posterior column (Fig. 50C.2). It is present in 2.7% of all traumatic SCIs (McKinley et al., 2007). Motor function is lost distal to the site of the injury. Spinothalamic function may be disrupted, leading to hyperesthesia and hypoalgesia below the level of the lesion. Though this syndrome is classically described for anterior spinal artery compromise, in the setting of trauma, it is due to flexion injuries or retropulsed disk or bone. Anterior cord syndrome carries a worse prognosis than other cord syndromes.
Posterior Column Syndrome
Posterior column syndrome is a rare condition with an incidence of less than 1%. This syndrome has been linked to neck hyperextension injuries. Injuries occur to the posterior aspect of the cord (Fig. 50C.3). Since the posterior columns are injured, there is usually a loss of position sense, with retained spinothalamic function. Motor function can be affected as well. Although this syndrome has been mentioned in the literature, it has been recently omitted from the International Standards for Neurological and Functional Classification of SCI (revised 2006) and is not currently recognized as a separate syndrome.
Brown-Séquard Syndrome
Brown-Séquard syndrome accounts for 1% to 4% of all traumatic SCIs. Injuries affect the lateral half of the cord (Fig. 50C.4). It occurs most frequently in the cervical spine and is usually due to penetrating injuries and (less commonly) blunt trauma including disk herniations. In cases of blunt trauma, Brown-Séquard syndrome usually occurs in the context of hyperextension injuries, though it has been observed in flexion injuries, locked facets, and compression-related injuries. Below the level of the lesion, it classically manifests with ipsilateral pyramidal deficit, loss of ipsilateral tactile discrimination, position sense, and vibratory sensation, and loss of pain and temperature sensation on the contralateral aspect of the body one to two dermatomes below the level of the injury.
There is rarely this classic presentation of Brown-Séquard syndrome, however. More frequently, patients presenting with Brown-Séquard syndrome present with a variation of the classic syndrome, termed Brown-Séquard plus (Taylor and Gleave, 1957). With Brown-Séquard plus, there is asymmetrical hemiplegia as well as hypalgesia more prominent on the less paretic side. Patients presenting with a clinical picture consistent with a classic Brown-Séquard syndrome injury have a worse prognosis than patients presenting with a variation of the syndrome, but the overall prognosis is good. Brown-Séquard has the best functional motor recovery when compared to other clinical spinal cord syndromes. Most subjects obtain bowel and bladder continence. Patient having predominantly more weakness in the upper extremities compared to the lower extremities have a favorable outcome in regard to ambulating. The symptoms of Brown-Séquard syndrome may appear instantaneously or in a delayed fashion. Furthermore, they may occur in conjunction with other spinal cord syndromes.
Conus Medullaris Syndrome
There is high probability that injuries to the thoracolumbar region can involve the conus medullaris. The conus medullaris represents the transition of the spinal cord from the central nervous system to the peripheral nervous system. The location of this region is highly variable—between the T12-L1 disk space to the middle third of L2 in the majority of the population (Fig. 50C.5). Thus, injury to the spinal column at the T12-L1 junction portrays inaccuracies regarding the exact injury to the neurological system. The lumbar parasympathetic fibers, sacral sympathetic fibers, and sacral somatic nerves originate in the conus medullaris. The classic presentation entails lower-extremity weakness, absent lower-limb reflexes, and saddle anesthesia. There is usually mixed upper motor neuron and lower motor neuron involvement. Loss of the bulbocavernosus and anal reflexes is permanent, differentiating conus medullaris syndrome from SCIs that have a return of these reflexes within 48 hours of the injury. Patients typically have an areflexic bowel and bladder (low-pressure, high-capacity bladder). The most common injuries to the vertebral column resulting in this condition are burst fractures or fracture-dislocation. There is no strong clinical evidence favoring surgical intervention over nonsurgical intervention for conus medullaris injuries. Furthermore, if surgical intervention is performed, there is no compelling evidence to suggest that earlier decompression affects functional outcome.
Cauda Equina Syndrome
The cauda equina is defined as the region of the neuroaxis occupied by the filum terminale. The only neurological structures in this region include the lumbar and sacral roots. Injuries in this location are typically a pure lower motor neuron injury (Fig. 50C.6). Findings often include absent bulbocavernosus reflex, absent deep tendon reflexes, flaccid urinary bladder, and reduced lower-extremity muscle tone. It is differentiated from conus medullaris syndrome by the presence of asymmetrical weakness and the absence of upper motor neuron involvement (Table 50C.2). Like conus medullaris syndrome, burst fracture and fracture-dislocation are the most common vertebral column injuries associated with this condition. Cauda equina injuries have better recoveries owing to the resiliency of the roots to injuries and the greater regeneration capacity of the roots compared to the spinal cord. The sacral roots, however, are very delicate, and injuries to them may be permanent. In general, cauda equina syndrome in the setting of herniated disk pathology is treated early (within 24 hours) if possible to prevent residual symptoms (Kennedy et al., 1999). Functional outcome in a traumatic setting, however, is similar to conus medullaris syndrome. There is no strong evidence correlating functional outcome to surgical decompression, nor is there any evidence that suggests cauda equina injuries fare better with early versus late decompression.
Table 50C.2 Similarities and Differences between Conus Medullaris Syndrome and Cauda Equina Syndrome
Conus Medullaris Syndrome | Cauda Equina Syndrome |
---|---|
Upper and lower motor neuron involvement | Lower motor neuron involvement |
Symmetrical motor impairment | Asymmetrical motor impairment |
Vertebral column injuries between T12-L2 | Vertebral column injuries distal to L2 |
Absent deep tendon reflexes | Absent deep tendon reflexes |
Permanent areflexic bladder | Permanent areflexic bladder |
Absent bulbocavernosus reflex | Absent bulbocavernosus reflex |
Transient Spinal Cord Syndromes
An estimated 7.3 out of 10,000 football participants suffer a cervical cord neuropraxia (Torg et al., 1997). Cervical cord neuropraxia is typically described as any motor or sensory complaints in any extremity lasting 15 to 30 minutes, but some cases can last up to 24 to 48 hours (Bailes, 2005). This injury is typically due to hyperextension, hyperflexion, or axial loading of the cervical spine. Cervical cord neuropraxia has been attributed to local anoxia and elevation of intracellular calcium (Torg et al., 1995). The description Bailes gave was “pathophysiologically similar to cerebral contusions, spinal cord concussion has become accepted to define those instances in which sufficient forces result in temporary inhibition of spinal cord impulse transmission without causing structural damage to the vertebral column or spinal cord, and is known to occur in athletes.” Bailes also concluded that “a single episode of temporary spinal cord dysfunction in an athlete with spinal stenosis will substantially increase the risk of future catastrophic SCI.” In his series, patients who returned to contact sport activities with no effacement of cerebrospinal fluid (CSF) around the cord or any radiographic abnormalities suggestive of cord damage encountered no further episodes of recurrent transient SCI with a mean follow-up period of 40 months.
Spinal Shock
Some characteristics of spinal shock have been noted. The severity of the injury correlates with the severity of spinal shock. An injury alters reflexes that occur closest to the insult first, with those more distal from the transection presenting later. Thus, high-level cervical injuries may have retention of sacral reflexes, such as a preserved bulbocavernosus and anal wink. The observation that a proximal-to-distal spread of reflex depression occurs on the order of minutes suggests a physiological explanation for these changes. It has been hypothesized that the loss of supraspinal input leading to hyperpolorization of neurons is responsible for this physiological change. There have been additional observations that an upward spread of reflex depression, the Schiff-Sherrington phenomenon, is not uncommon. It is important to delineate blood pressure drops from circulatory shocks from those of spinal shock (Table 50C.3). As there is loss of sympathetic tone, there is pooling of blood in the venous system and a loss of sympathetic tone in the cardiovascular system. On the one hand, circulatory shock requires volume replacement, and on the other hand, spinal shock requires vasopressors. As spinal shock resolves, muscle spindle reflexes return in a caudal-to-cranial direction, except at the level of injury. Over time, a spastic syndrome results.
Table 50C.3 Similarities and Differences between Neurogenic and Hypovolemic Shock
Neurogenic Shock | Hypovolemic Shock |
---|---|
Hypotension | Hypotension |
Bradycardia | Tachycardia |
Areflexia | Normal reflexes |
Responsive to pressors | Responsive to volume replacement |
There is no uniform consensus on what constitutes the cessation of spinal shock. Most references define the end of spinal shock with a return of certain reflexes. However, not all reflexes are uniformly depressed in each patient; reflexic changes are individualized. The resolution of spinal shock occurs over a period of days to months, so there is a slow transition from spinal shock to spasticity that occurs on a continuum. It has been proposed that this transition comprises four phases (Ditunno et al., 2004). The first phase occurs from 0 to 24 hours following the injury and is characterized by areflexia or hyporeflexia. Deep tendon reflexes are absent. During this period, the first pathological reflex to appear is the delayed plantar reflex, followed by a series of cutaneous reflexes such as the bulbocavernosus, abdominal wall, and cremasteric reflex. Impaired sympathetic control can lead to bradyarrhythmias, atrioventricular conduction block, and hypotension. Motor neuron hyperpolarization explains the changes that occur. Phase 2 occurs between day 1 and day 3 post injury. Cutaneous reflexes are more prominent during this period, but deep tendon reflexes remain mute. It is not unusual for elderly individuals and children to experience recovery of deep tendon reflexes during this time. The Babinski sign may become apparent in the elderly as well. Denervation supersensitivity and receptor up-regulation account for these changes in the second phase. The next phase occurs between 4 days to 1 month post injury. Deep tendon reflexes usually recuperate by day 30. There is great disagreement about when these reflexes appear. The recovery of the Babinski response closely parallels the return of the ankle jerk reflex. There is also diminution of the delayed plantar reflex. Autonomic changes such as bradyarrhythmias and hypotension begin to subside. This time period is reflected by axon-supported synapse growth. The fourth phase is dominated by hyperactive reflexes and occurs from 1 to 12 months after injury. Vasovagal hypotension and bradycardia generally resolve in 3 to 6 weeks, but orthostatic hypotension may take 10 to 12 weeks before it disappears. Episodes of malignant hypertension or autonomic dysreflexia begin to appear during this time period. Soma-supported synapse growth accounts for these findings.
Mechanisms and Types of Injuries
Cervical Spine Fractures
Atlanto-Occipital Dissociation
Atlanto-occipital dissociations occur from high-energy impact and frequently lead to death. When the diagnosis is missed, subjects can have poor outcomes. These injuries result in laceration of the pontomedullary or spinomedullary junctions and are more common in children because of the horizontal orientation of their atlanto-occipital joint. Atlanto-occipital dissociations are classified into three types based on the dislocation of the condyles in relation to the atlas: type I, anterior; type II, vertical; and type III, posterior (Traynelis et al., 1986). Patients who survive this injury can present with cranial neuropathy or weakness. The diagnosis in survivors can be made by measuring the dislocation on lateral cervical x-rays. Additional findings on x-rays include prevertebral soft-tissue swelling. However, studies with computed tomography (CT) and MRI are recommended in patients with suspected atlanto-occipital dissociation. When craniocervical subarachnoid blood is present, atlanto-occipital dissociation should be suspected. The diagnosis of this injury requires prompt reduction and stabilization in a halo vest, followed by fixation by occipital-cervical fusion. Traction can cause further deterioration and should be avoided.
Occipital Condyle Fractures
Occipital condyle fractures were first described by Bell in 1817 but have been more frequently diagnosed in head injuries since the introduction of CT. They tend to occur with high-energy compression shear forces. The frequency of occipital condyle fractures has been reported as high as 16.4% of patients who undergo high-energy blunt craniocervical trauma (Bloom et al., 1997). Patients can present with a complaint of subtle neck discomfort, but when associated with traumatic brain injury or atlanto-occipital dissociation, more severe presentation may occur. Thus, the variety of presentations seen include low Glasgow Comma Scale values, retropharyngeal soft-tissue swelling, occipitocervical tenderness, reduced craniocervical motion, and lower cranial neuropathy. Before the CT scan, the sensitivity of detection by plain radiography was approximately 3.2% (Hadley et al., 2002).
The classification described by Anderson and Montesano (1988) is still widely used today. According to their classification, there are three types of occipital condyle fractures: (1) a unilateral impacted fracture resulting in comminuted elements, (2) a linear basilar skull fracture that extends into the condyle, and (3) avulsion fractures of the condyle. Type III injuries warrant a high degree of caution, as they may have associated atlanto-occipital dissociation and can bear instability with alar and tectorial membrane disruption. For this reason, they are treated more aggressively with halo immobilization followed by occipital-cervical stabilization. External mobilization should be considered for type I and II injuries.
Atlantoaxial Injuries
Fractures of the axis occur in the odontoid process, pars interarticularis, vertebral body, lateral masses, or spinous process. Vertebral body and spinous process fractures are conservatively treated with external immobilization. The most common injuries to C2 involve the odontoid process. Injuries to the spinal cord result in instability from translational displacement of C1 on C2. Treatment strategies involve preserving axial rotation of the neck, which can be up to 60 degrees at this level. The most widely used classification of odontoid fractures is the Anderson and D’Alonzo classification. Type I fractures project into the upper portion of the odontoid. Type II fractures involve the base of the odontoid. A subgroup of these fractures is known as type IIA, which is a comminuted fracture at the base of the odontoid with associated free fragments. Type III fractures descend into the vertebral body. Type I and type III odontoid fractures are treated with external rigid immobilization with cervical collar for 8 to 12 weeks. There is less blood supply at the base of the odontoid, which factors into lower fusion rates and avascular necrosis than either type I or type III fractures. Furthermore, odontoid fractures occurring in patients older than 50 years of age have a 21-fold risk of nonunion (Lennarson et al., 2000). Thus, strong consideration should given to treating patients with type II odontoid fractures with halo bracing for 8 to 12 weeks. If no fusion is seen, surgical stabilization is necessary. For patients with type II fractures who are older than 50 years of age and have displacement greater than 5 mm, or with a comminuted component at the base of the odontoid (type IIA), surgical stabilization should be considered as the first treatment option. Usually, early surgical treatment with an odontoid screw can preserve C1/C2 rotation. Surgical stabilization following failed nonunion of odontoid fractures with nonoperative treatment requires a C1/C2 fusion limiting rotation.
Subaxial Cervical Spine Injuries
Flexion-compression injuries are most often due to ventral axial loading. The prototypical cause of this injury is the classic diving injury. As a result of the forces directed on the body, there are compressive fractures seen in the anterior vertebral body. Posterior element fractures can occur in up to 50% of cases. There can be mild distraction of the facets and disruption of the posterior ligaments. With intact facets, these injuries are stable and can be treated with external immobilization. Vertical compression fractures lead to burst fractures. Surgery is warranted for retropulsion of bony elements into the canal leading to neurological deficits. Mechanical stability must be assessed for each of these cases. Teardrop fractures represent the extreme variant of these injuries and result from severe hyperflexion and axial forces. They appear as a fractured vertebral body with associated retrolisthesis and posterior ligamentous disruption and dislocation. These are highly unstable fractures and require surgical stabilization. Flexion-distraction injuries typically involve minimal osseous injury, with a predominance of ligamentous injury. They vary in severity from hyperflexion strain to bilateral jumped facets. Thus, MRI can typically be used to evaluate the extent of these injuries that is not obvious on lateral C-spine x-rays. A significant number of subjects who are diagnosed with jumped facets have neurological deficits; 21% of patients diagnosed with unilateral jumped facets are neurologically intact (Shapiro, 1993), compared to only 10% of patients diagnosed with bilateral jumped facets (Wolf et al., 1991). Treatment of locked facets begins with attempts at closed reduction with traction. Failed attempts warrant an open reduction. Once reduced, stabilization is required, with surgical fixation being the preferred method. Compressive-extension injuries can cause vertebral arch and laminar fractures and may lead to instability and a need for surgical stabilization. Extension-distraction injuries are common in falls and can produce central cord syndrome in the setting of trauma in an elderly patient with baseline cervical spondylosis. No fractures or ligamentous injuries may be present, but buckling of the ligamentum flavum is sufficient to damage the cord. Surgical decompression is beneficial to these patients, but the timing of such decompression is controversial. Lateral flexion injuries may lead to unilateral vertebral body or posterior arch injuries. They are usually stable and can be treated with external immobilization.
Thoracolumbar Injuries
In general, the thoracic and lumbar spine is divided into three segments: an anterior column, middle column, and posterior column. The anterior column extends from the anterior longitudinal ligament to the middle of the vertebral body. The middle column is defined as the portion between the middle of the vertebral body to the posterior longitudinal ligament. The posterior column is the remaining extent of the vertebrae. The classification of thoracolumbar fractures utilizes the three-column model of the spine. A CT scan can provide precise information regarding the extent of injury. There are four major categories of thoracolumbar spine fractures (Fig. 50C.7). Compression fractures involve compression of the anterior body, leading to wedging. Compression of the anterior and middle column is seen in burst fractures. With burst fractures, radiographic indications for surgery are typically loss of vertebral height of 50%, 30 degrees of kyphosis, or 50% canal compromise from retropulsion of elements. There may be neurological deficits associated with retropulsion. Seat belt injuries involve the middle and posterior column. Patients are generally neurologically intact. However, these injuries are typically deemed unstable fractures and should be treated with surgical stabilization. In fracture-dislocation injuries, involvement of all three columns is seen. The radiographic appearance of these injuries suggests a flexion-rotation, sheer, or flexion-distraction mechanism. These fractures are highly unstable and require surgical intervention.
Penetrating Spinal Cord Injuries
The majority of penetrating SCIs are due to gunshot wounds to the spine and second only to automobile accidents in causing spinal cord–related disability. Stab wounds to the spine are less commonly seen and present with Brown-Séquard features. When making an evaluation of gunshot injuries to the spine, the trajectory of the bullet must be considered in addition to the physical location of the bullet. It is important to assess whether bowel penetration and contamination is present. The destructive nature of a bullet is related to direct injury from the bullet itself, the shock waves it creates, and temporary cavitation. These factors are dependent on the size and velocity of the missile. On examination, injuries can present one level higher than the observed location of the bullet. CT scan can assess for instability and be more helpful than MRI, which can also be safely done. MRI will not typically influence acute treatment decisions unless an evolving hematoma is present. There has been debate over whether patients benefit from laminectomy and bullet removal with incomplete injuries. The National Institute of Disability and Rehabilitation Research suggests that there is no benefit from such heroic measures. However, patients with incomplete injuries with neurological deterioration should undergo decompression. The other indication for decompression includes injuries located at or below the level of the conus. Spinal nerve roots have a greater capacity to recover after decompression. There are cases of late neurological deterioration occurring as late as 17 years post injury (Ajmal et al., 2009). In these cases, improvement of symptoms can be seen after excision of the bullet and the surrounding reactive tissue. Spinal instability should warrant surgical stabilization. Infection can be common with a contaminated bullet causing viscus perforation and entering the spine. In a civilian population, long-term antibiotics (2-week course) have been shown to have favorable outcomes (Roffi et al., 1989). Steroids should be avoided for penetrating spine injuries.
Management of Acute Spinal Cord Injuries
Radiographic Evaluation
Plain Radiography
Initial radiographic evaluation of the spine should be done with plain x-rays. The decision to obtain cervical spine imaging is based on the NEXUS (National Emergency X-Radiography Utilization Study) criteria (Hoffman et al., 2000), a set of five screening assessments created to help guide physicians in making a decision to exclude low-risk/low-yield patients from undergoing cervical radiography. Patients meeting all the criteria in Box 50C.2 can bypass imaging. This study reported a 99% sensitivity and a 12.9% specificity in diagnosing SCIs when using these criteria. More recently, the Canadian C-Spine Rule was noted to be superior to the NEXUS criteria in terms of sensitivity and specificity for alert, stable patients in whom cervical spine injury is a concern (Stiell et al., 2003). Screening criteria include high-risk factors, low-risk factors, and ability to actively rotate the neck. Using the Canadian C-spine Rule, patients older than age 65 who are subject to a “dangerous mechanism” or experience paresthesias (high-risk criteria) should receive plain radiography. Those not meeting any of the high-risk criteria are assessed further. If this subgroup of patients have delayed pain in the neck, posterior neck tenderness, cannot tolerate sitting or ambulatory position at any time after injury, or are involved in an accident that is more than a simple rear-end motor vehicle collision (low-risk criteria), then imaging should be obtained. If they lack any of the listed findings, they are further assessed for their ability to rotate the head. Plain radiography should be obtained for patients incapable of rotating their head 45 degrees in either direction.
Typical plain radiography of the cervical spine should include anteroposterior (AP), open odontoid, and lateral views with flexion-extension. An adequate lateral C-spine x-ray should visualize the area between the occiput and the top of T1. A so-called swimmer’s view may be helpful to view the caudal portion of the cervical spine. Four lines should be drawn on a lateral C-spine x-ray to evaluate for subluxation or fractures: (1) anterior vertebral body line, (2) posterior vertebral body line, (3) spinal laminar line, and (4) posterior spinous line (Fig. 50C.8). Findings showing more than 3.5 mm of subluxation or kyphotic angulation greater than 11 degrees to adjacent vertebral body segments can imply instability. Subluxation on the order of 25% or 50%, respectively, suggests unilateral or bilateral jumped facets. Mild flexion distraction injuries can be suggested by enlargement of the interspinous distance. The atlantodental interval (ADI) is a measure taken from the anterior margin of the dens to the closest portion of the anterior arch of C1. A value greater than 3 mm can suggest transverse ligament disruption. The Powers ratio is defined as a ratio of the distance from the basion to the posterior arch of the atlas divided by the distance from the opisthion to the anterior arch of the atlas. Atlanto-occipital dissociation can be suggested with a Powers ratio greater than 1. Prevertebral soft-tissue swelling should also be noted on the lateral C-spine x-rays. The upper limit of normal at the level of C3 is 4 mm. The amount of lateral mass overhang of C1 on C2 seen on the odontoid views can be measured to assess the integrity of the transverse ligament. A sum of lateral mass overhang greater than 7 mm is suggestive of transverse ligament disruption.
Complete x-rays of the whole spine should performed if any abnormalities of the spine are detected on imaging, since noncontiguous spine injuries are seen in 10.5% of cases (Vaccaro et al., 1992). Plain radiographs of the thoracic and lumbar spine should include AP and lateral films to assess for alignment, kyphosis, disk height, and fractures. Thoracic fractures can be missed on x-rays and may require a CT or MRI if injury is suspected in this region. Scoliosis films can detect present deformity and sagittal imbalance but more importantly can be used as a baseline study for assessing progressive posttraumatic kyphosis.
SCIWORA and SCIWORET
Spinal cord injuries without radiographic abnormalities (SCIWORA) were first diagnosed in 1982 before the MRI era (Pang and Wilberger, 1982). Since the advent of MRI, there has been a broad spectrum of injuries present in SCIWORA patients that range from normal MRI imaging to complete cord disruption or abnormal disk pathology. SCIWORA are more commonly encountered in the pediatric population. The incidence of SCIWORA in cases of traumatic myelopathy of children between 1 and 17 years of age has been estimated at 34.8% (Pang, 2004). Reasons to suggest a higher incidence in the pediatric population center around the laxity of ligaments, expandability of the intervertebral disk, and the biological and anatomical differences noted in the spine between children and adults. Other considerations include the proportionally larger size of children’s heads and the lack of development of paravertebral muscles. Since the advent of the MRI, the definition of SCIWORA has been revised to exclude compressive lesions found on MRI, but not intraneuronal lesions. Some practitioners feel that any spinal cord lesions should be excluded in the definition. The acronym SCIWORET for spinal cord injury without radiographic evidence of trauma has recently been seen in the context of medical literature. SCIWORET was intended to refer to SCI without evidence of bony or ligamentous disruption in adults with baseline spondylotic changes, typically presenting with central cord syndrome. Thus, the definition of SCIWORA has been ambiguous depending on the strictness of the inclusion criteria.
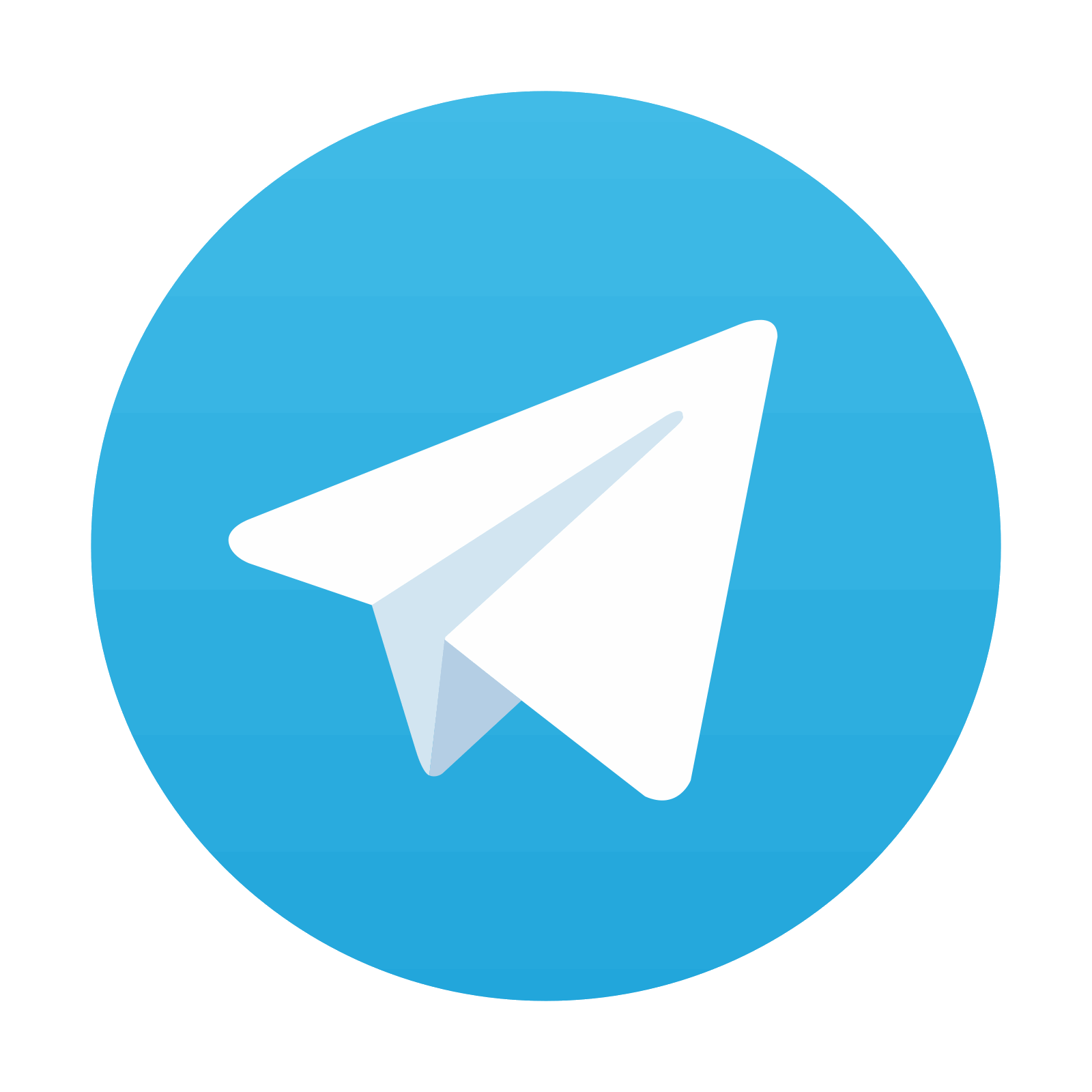