html xmlns=”http://www.w3.org/1999/xhtml” xmlns:mml=”http://www.w3.org/1998/Math/MathML” xmlns:epub=”http://www.idpf.org/2007/ops”>
The prevailing view that cortical gray matter is the seat of higher functions has inspired a vast amount of research, and similar productivity can be imagined when white matter is considered with the same degree of interest. Many new directions can be imagined from thinking about the role of white matter in normal cognition, all of which implicate the pursuit of a better understanding of white matter as it participates in the architecture of cognitive operations. First, the normal neuroanatomy of myelinated systems in the brain is a topic of vigorous investigation. Next, the study of white matter connectivity in distributed neural networks is attracting steady interest as the operations of these networks become more securely understood. The contribution of cerebellar white matter to cognition merits discussion as support grows for the provocative idea that the cerebellum has neurobehavioral importance. Finally, the emerging role of inflammation as a general pathological phenomenon within white matter deserves comment.
Normal white matter anatomy
An important outcome of work on myelinated systems is that the normal white matter of the brain will be much better understood. Classic neuroanatomy, for all its elegance and detail, has left many questions unanswered about the origin, course, and termination of white matter tracts (Schmahmann and Pandya, 2006), especially for clinicians attempting to assign functions to specific connecting structures. Indeed, neurologists at present are accustomed to conceptualizing the white matter as an essentially homogeneous mass of myelinated tissue packed into large bundles that cannot be readily teased out one from another. New neuroimaging methods promise to address this issue with increasing sophistication. The steady improvement in technology, notably including increases in field strength of magnets used for magnetic resonance imaging (MRI), will also help clarify the nascent topic of intracortical myelin, long known from neuroanatomy but only now visible in vivo (Schmahmann and Pandya, 2006; Nieuwenhuys, 2013). At the same time, traditional neuroanatomic studies can be informed by clinical neuroimaging data, and useful insights will come forth whether the neuroimaging findings confirm the currently accepted neuroanatomy or present new information. A productive interplay can be developed between neuroimaging and neuroanatomy to refine knowledge of normal white matter (Schmahmann and Pandya, 2006; Mesulam, 2012; Catani et al., 2012a).
An instructive example of the application of combined neuroimaging and neuroanatomy can be seen in the recent discovery of the frontal aslant tract (Catani et al., 2012b). This short frontal connection, which links the supplementary motor area with Broca’s area, was identified with MRI and then confirmed by postmortem dissection (Catani et al., 2012b). Subsequent study of patients with primary progressive aphasia demonstrated the importance of this tract in verbal fluency and grammar processing (Catani et al., 2013a). The recognition of the frontal aslant tract also clarifies the understanding of a related syndrome, transcortical motor aphasia, which was thought on the basis of computed tomography studies to involve a disruption of the connections between the supplementary motor area and the left perisylvian region (Freedman, Alexander, and Naeser, 1984). Thus new research on white matter anatomy confirmed with more advanced technology what had been previously suspected on clinical grounds.
The discovery of the frontal aslant tract exemplified how improved understanding of normal white matter anatomy will generate a more informed approach to the identification and interpretation of white matter lesions seen in clinical settings. Neurologists are well aware that it is at times not a trivial task to determine whether a given brain structure seen on neuroimaging is normal or abnormal. Knowledge of normal tract anatomy will enhance the recognition of what white matter structure is indeed not normal. White matter tractography appears to be among the most exciting clinical applications of advanced neuroimaging, and diffusion tensor imaging (DTI) holds the most promise. In the tradition of Geschwind, DTI promises to expand the understanding of disconnection syndromes by directly demonstrating damaged tracts in vivo that can be implicated in a variety of neurobehavioral syndromes (Catani, 2006).
With further progress, it is not unduly fanciful to imagine that tractography of tracts large and small will soon be applied in the routine clinical practice of neurology and psychiatry. A general consensus holds that MRI scanners with higher magnetic field strength will be crucial in the evolution of this research (Filippi et al., 2014), and other technical advances can safely be assumed to appear in the near future. As the location, size, extent, degree of myelination, sites of origin and termination, and functional affiliations of specific tracts become more clear, lesions of individual tracts and in multiple tracts will be identifiable by noninvasive methods. Early and accurate identification of these lesions may allow prompt and effective treatment that can potentially avert the development of white matter dementia (WMD) or its predecessor, mild cognitive dysfunction (MCD).
A research topic that is likely to impact treatment is the role of white matter lesions in neurochemical systems relevant to cognition (Butt, Fern, and Matute, 2014). A number of neurotransmitters are found in the white matter, not only within the large tracts but also within the smaller fascicles of the cerebral cortex and deep gray matter (Butt, Fern, and Matute, 2014). Box 12.1 displays the major white matter neurotransmitters currently recognized. This topic is broad and largely beyond the scope of this book, but two implications are immediately apparent.
Cholinergic
Glutamatergic
Dopaminergic
Serotonergic
Adrenergic
GABAergic
Glycinergic
Purinergic
First, because many neurotransmitters course through axons that emanate from subcortical neuronal cell bodies and ascend to the cortex, they are susceptible to disruption by a variery of white matter lesions. In this regard, an instructive example is the cholinergic system (Román, 2005). Acetylcholine is an essential neurotransmitter conveyed by projections from the basal forebrain to widespread areas of the cerebrum, and is involved in many aspects of attention and memory. Neuroanatomic studies have found that cholinergic projections course within areas of white matter that are vulnerable to white matter disorders (Selden et al., 1998), including leukoaraiosis (Swartz, Sahlas, and Black, 2003) and cerebral autosomal-dominant arteriopathy with subcortical infarcts and leukoencephalopathy (CADASIL; Mesulam, Siddique, and Cohen, 2003). It is therefore plausible that cholinergic augmentation may come to occupy an important position in the treatment of WMD and MCD. Such an approach is well known in the treatment of patients with Alzheimer’s Disease (AD), but in this disease the cholinergic deficit results from neuronal loss in the basal forebrain and not because of damage to cholinergic fibers extending rostrally (Kim, Moon, and Han, 2013). Extension of this generic concept to other neurotransmtters coursing within the white matter may offer a wide range of pharmacological treatment opportunities.
Second, because white matter is characterized by the absence of neuronal cell bodies and traditional synapses, and neuron-to-neuron communication does not occur, white matter neurotransmitters are engaged in other forms of signaling such as axo-oligodendroglial transmission (Butt, Fern, and Matute, 2014). This phenomenon in turn contributes to white matter plasticity (Fields, 2010), as has been considered earlier and will be further discussed in the next chapter.
Other questions germane to the normal anatomy of white matter are also relevant. Although the large brain of Homo sapiens has acquired a substantial complement of myelinated axons that permits efficient signaling across large intracranial distances (Zhang and Sejnowski, 2000), a percentage of the cerebral axons is unmyelinated. In the rat, an estimate of 80% has been offered as the percentage of corpus callosum axons that is unmyelinated (Gravel, Sasseville, and Hawkes, 1990), suggesting that human brains may harbor a proportion of similar axons. Preliminary autopsy data from the human fornix indicate that myelinated fibers outnumber those that are unmyelinated (Ozdogmus et al., 2009), but a substantial percentage of human brain axons is likely to be found devoid of myelin. The function of these fibers, however, is not well understood, and their importance for neurobehavioral competence is similarly obscure. Some evidence exists for a selective vulnerability of unmyelinated axons to trauma, possibly because they lack the structural support and protection of circumferential myelin (Reeves et al., 2012). More data on the location, relative abundance, location, functional role, and specific vulnerability – or resistance – of these axons to disease, injury, or intoxication will prove illuminating.
Another area deserving investigation is the presence of myelinated fascicles in all layers of the cerebral cortex. The accomplishments of the Vogts in the early twentieth century as they labored with autopsied human brains to describe a myeloarchitecture of the cortex (Chapter 3) can provide a basis for similar studies today, but now with the assistance of powerful in vivo neuroimaging. Intriguing ideas are being advanced, including the somewhat counterintuitive notion that increased intracortical myelination – such as that found in the line of Gennari within the calcarine cortex – may actually inhibit synaptic plasticity (Glasser et al., 2014). Myelin in the cortex may thus serve a much different purpose than myelin elsewhere in the brain. Evidence now suggests that heavily myelinated sensorimotor cortices function to enhance highly evolved but relatively invariant sensory and motor activity, whereas lightly myelinated association cortices – which have greatly expanded in humans over evolution compared to higher primates – subserve critical higher functions that require more flexibility and hence synaptic plasticity (Glasser et al., 2014). Thus the possibility presents itself that myelin in large subcortical tracts enhances cognition by facilitating information transfer between gray matter regions within distributed neural networks, whereas myelin within the cortex has the opposite effect, and is in fact more useful in its absence for the optimal function of the cortex in cognitive operations.
Intracortical myelin also appears to play a role in the recently introduced effort to measure cortical thickness as a method of studying aging and dementia (Teipel et al., 2008). In the continuing search to find reliable biomarkers of various dementias, the assessment of cortical thickness has become a popular application of rapidly emerging neuroimaging technology. While synaptic and neuronal loss likely plays a major role in any reduction in cortical thickness observed in older individuals, age-related changes in gray matter volume are also thought to result in part from a decline in intracortical myelin (Kochunov et al., 2011). Recent MRI studies using a 7.0 T magnet in normal humans have succeeded in visualizing white matter fascicles within the auditory cortices (De Martino et al., 2014), an encouraging development that could lead to high-resolution imaging of intracortical myelin damage. The mechanisms and implications of myelin loss within the cortex are provocative but little understood, yet the possibility of a parallel decline in myelin within large tracts and in the cortex raises interesting questions about the processes of aging as well as many dementia syndromes.
White matter in distributed neural networks
Neuroanatomic investigations will in turn clarify the architecture of distributed neural networks, the central organizational feature of the brain as it subserves the wide array of cognitive and emotional operations that make up the human behavioral repertoire (Geschwind, 1965; Mesulam, 1990; Solar and Stoner, 2011). White matter is the connecting tissue between cortical and subcortical gray matter regions within and between the hemispheres, and recent data also point to the importance of white matter in networks involving the cerebellum (Schmahmann and Pandya, 2006). The concept of the connectome – “a comprehensive structural description of the network of elements and connections forming the human brain” – has recently been proposed to capture the spirit of this endeavor (Sporns, 2011; Van Essen et al., 2013), and mapping of human brain connectivity is underway (Toga et al., 2012; Ajilore et al., 2013; DiMartino et al., 2014). Large tracts, intracortical myelin, and white matter fascicles coursing through deep gray matter nuclei all warrant study.
In the practice of behavioral neurology as well as a host of research endeavors, distributed neural networks already serve to inform thinking on brain–behavior relationships. For the past 200 years, the localization of cognitive function has been a central interest of neuroscience, beginning with pioneering studies of nineteenth-century neuroscientists who first began considering the idea that specific functions could be associated with discrete regions of the brain (Young, 1970). The first notable figure of this period was Franz Joseph Gall, an accomplished neuroanatomist who is unfortunately better known today as the progenitor of the discredited notion of phrenology. Before venturing with his colleague Johann Kaspar Spurzheim into the speculations of phrenology, which held that various mental faculties could be determined by palpation of bony irregularities of the skull that signified underlying brain areas, Gall conducted pioneering neuroanatomic studies that were far ahead of his time. Using dissections of human brains, Gall and Spurzheim emphasized the importance of the cerebral cortex in the organization of brain functions, and recognized the functional specialization of cortical regions (Schmahmann and Pandya, 2006). In addition, they identified white matter tracts linking cortical regions, dividing these tracts into projection and association fibers, a classification still in use today (Schmahmann and Pandya, 2006). Gall is thus a key figure in the history of systems neuroscience (Schmahmann and Pandya, 2006), and even the practice of phrenology can be interpreted as a flawed but influential harbinger of more legitimate brain–behavior correlations that would subsequently appear. Later in the nineteenth century came seminal observations on aphasia by Paul Broca (1861) and Karl Wernicke (1874), who not only established the foundation for the cortical localization of language but also introduced white matter connectivity as an integral component of the organization of language.
As time went on, the idea of distributed networks steadily gained ground as it was appreciated that a slavish one gyrus–one function correspondence was not supported by the accumulating data. The operations of cognition and emotion came to be seen as both localized and distributed, so that the behavior resulting from brain activity was widely represented in cerebral networks (Mesulam, 1990). Much of the knowledge gained in this endeavor was derived from the monkey, but the limitations of studying this animal as a model of human connectivity are apparent (Mesulam, 2012). However, from the study of human diseases and the application of advanced neuroimaging, a number of distributed neural networks were tentatively established (Mesulam, 1990; Catani et al., 2012a), all presumed to involve combinations of gray and white matter structures (Filley, 2012). These networks (Table 12.1) include one for arousal involving the rostral brain stem, thalamus, and cerebral cortex (Parvizi and Damasio, 2001), the medial temporal lobe–hippocampal network for declarative memory (Jang and Kwon, 2014), a spatial attention network with major hubs in the right parietal and frontal lobes (Bartolomeo, Thiebaut de Schotten, and Chica, 2012), a visuospatial network with right parietofrontal distribution (Parks and Madden, 2013), a visual recognition network involving bilateral occipitotemporal regions (Tavor et al., 2014), an executive function network with frontal and parietal hubs (Gold et al., 2010), the limbic system as it subserves basic aspects of emotions and personality (Catani, Dell’acqua, and Thiebaut de Schotten, 2013b), and, as was recognized with the use of functional neuroimaging including positron emission tomography (PET) and functional MRI (fMRI), the salience network mediating social cognition (Seeley et al., 2007) and the default mode network subserving resting brain activity (Raichle et al., 2001). As functional neuroimaging began to explore connectivity using coactivation of cortical regions, it was found, reassuringly, that structural connectivity generally mirrors that which is found using studies of cortical function (Damoiseaux and Greicius, 2009).
Domain | Gray matter structures | Connecting tracts |
---|---|---|
Arousal | Reticular activating system | Medial forebrain bundle |
Thalamus | Thalamocortical radiations | |
Cerebral cortex | ||
Spatial attention | Parietal lobe (right) | Superior occipitofrontal fasciculus (right) |
Prefrontal cortex (right) | ||
Cingulate gyrus (right) | Cingulum (right) | |
Memory | Hippocampus | Fornix |
Diencephalon | Mammillothalamic tract | |
Basal forebrain | ||
Language | Broca’s area | Arcuate fasciculus |
Wernicke’s area | Extreme capsule | |
Visuospatial ability | Parietal lobe (right) | Superior occipitofrontal fasciculus (right) |
Frontal lobe (right) | ||
Visual recognition | Temporal lobes | Inferior occipitofrontal fasciculus |
Occipital lobes | ||
Executive function | Dorsolateral prefrontal cortices | Superior occipitofrontal fasciculus |
Parietal cortices | ||
Emotions and personality | Temporolimbic system | Medial forebrain bundle |
Orbitofrontal cortices | Uncinate fasciculus | |
Social cognition (salience network) | Anterior insula | Inferior occipitofrontal fasciculus |
Orbitofrontal cortex | ||
Anterior cingulate | Cingulum | |
Resting state (default mode network) | Anterior cingulate | Cingulum |
Posterior cingulate | ||
Precuneus | ||
Medial temporal cortices |
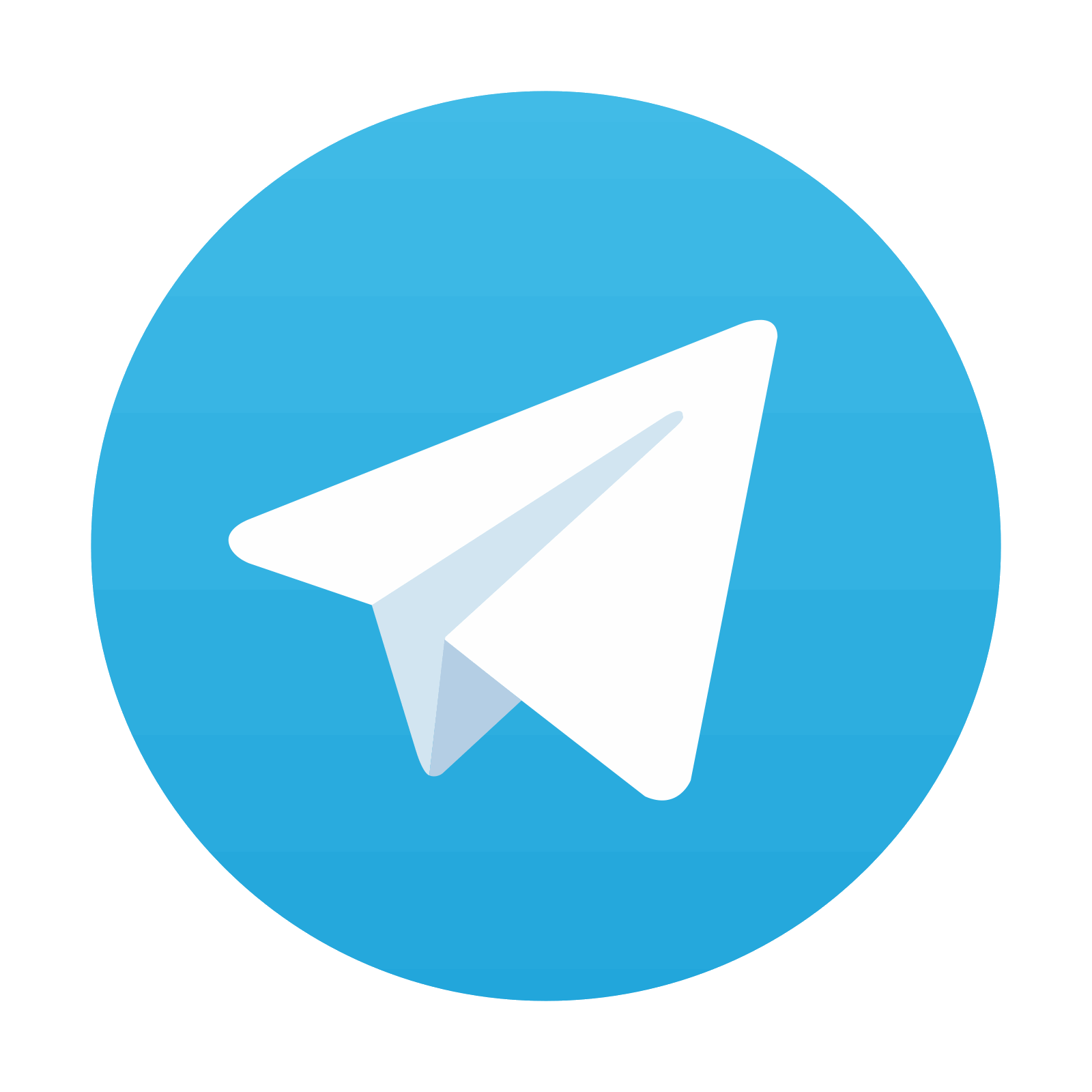
Stay updated, free articles. Join our Telegram channel
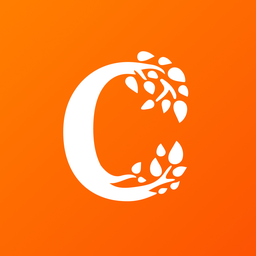
Full access? Get Clinical Tree
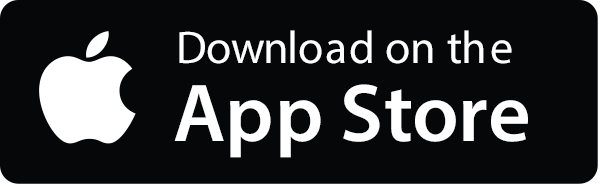
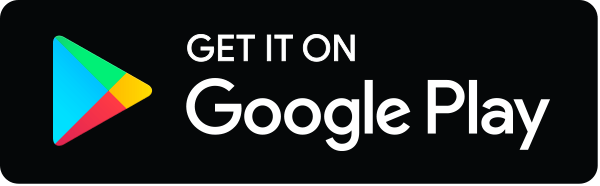