The coronal sectioning of 3-D SD-OCT data showed the seemingly random noise below the retina in cross-sectional images to be choroidal vasculature. (A) C-mode image of choroidal vasculature, which was generated by summing up the information within the slab of thickness indicated by the three horizontal light blue lines shown on the cross-sectional image (B).
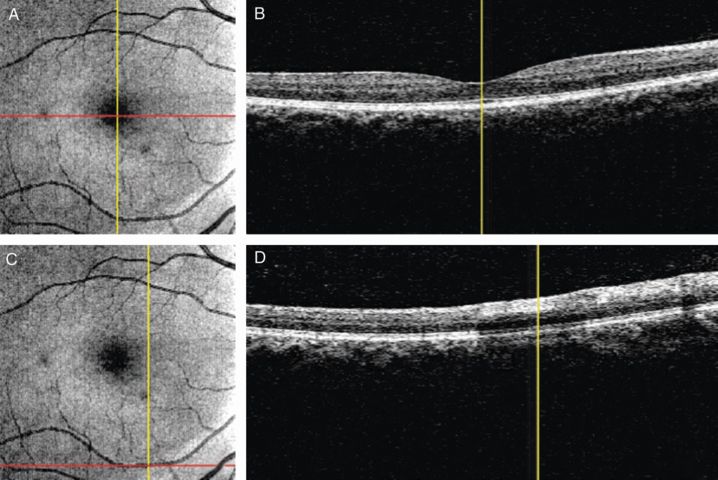
The optical coherence tomography (OCT) fundus image (summed coronal view of the 3-D OCT data), as shown in (A) and (C), permits pinpointing of the sampling location within the 3-D cube OCT data. Red lines on the fundus image indicate where the corresponding cross-sectional images on the right were sampled. The vertical yellow lines point the same location in the corresponding fundus and cross-sectional images. In (B), the vertical yellow line points out the position of the foveola, while in (D) it indicates the location of the blood vessel.
The transition to SD-OCT also changed how the OCT technology was commercialized. With the apparent public domain nature of the SD-OCT technology, multiple companies jumped into the medical OCT market. Currently, there are at least nine companies manufacturing SD-OCT. This competition accelerates technological improvement and innovation in a very positive way, as well as driving prices down for consumers.
In this chapter, many new technological advancements in the ocular OCT imaging field are discussed. Some are still in purely experimental stages, but others are on the verge of clinical commercialization or even already on the market. All provide particular advantages in a variety of aspects of OCT imaging that are useful for both clinical and research purposes.
Eye-tracking system
Eye movement artifacts on OCT images have been one of the biggest problems that affect both qualitative and quantitative assessment from the very beginning. When SD-OCT was commercialized, we hoped that the faster scanning speed of SD-OCTs would have solved the eye movement artifact problem. Ironically, the faster speed was used to increase sampling density (from 768 samplings on six radial 2-D slices to 40,000 samplings on a 3-D cube covering 6 × 6 mm area for approximately the same scan time of 1.2 to 1.4 seconds) instead of decreasing scanning time, resulting in the same eye movement artifacts in SD-OCT exhibited with TD-OCT (Figure 19.3). On the other hand, it is easier to catch such artifacts on SD-OCT than TD-OCT because the 3-D scanning allows an en face view of the image. Also, eye movements including micro-saccades are so fast that even near instantaneous scanning might still demonstrate some eye movement artifacts, although the magnitude becomes small with extremely fast scanning. Therefore, it seems common sense to add eye tracking to OCT.
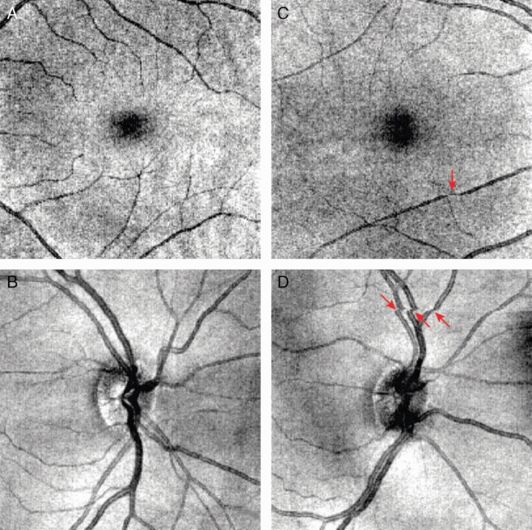
Eye motion artifacts during scanning. (A) and (C) present good scans, without eye motion artifacts or blinking, of macular region. (B) and (D) show scans with eye movement during the scans, as indicated by the red arrows. Eye motion artifacts can be observed as the discontinuity of the blood vessels on the fundus images and significantly affect the analysis results.
Eye-tracking OCT is not really a new technological advancement as of June 2014, because there are some commercial SD-OCT devices that already have this feature. They send two beams of light to the target eye simultaneously: one beam for tracking and the other beam for OCT scanning. With eye tracking, it not only resolves eye movement or blink artifacts occurring during scanning, but also enables repeated scanning at the same location. This allows signal averaging to improve the signal-to-noise ratio, resulting in greater image quality (Figure 19.4) [1–5]. It is also possible to register the scanning location to the previous scan so that scan-to-scan measurement variability can be minimized. This is particularly useful for longitudinal study to assess changes in a given subject [6].
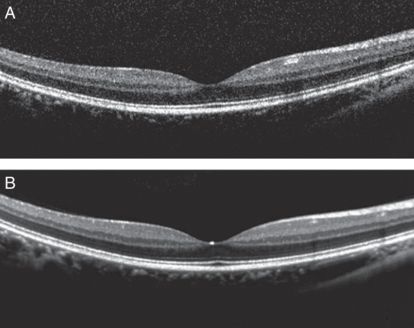
Cross-sectional images through the fovea from the same eye using OCT device with an eye-tracking system. (A) no frame averaging is applied. (B) 100 frames averaged. 100-frame averaged scan reveals stronger and clearer definition of the retinal layer structures with less speckle noise compared to a non-frame-averaged scan.
The one practical drawback of eye-tracking OCT is its prolonged scanning time. The fast eye movement and blinking during scanning forces the machine to discard the frame just taken and simply repeat scanning at the same location until there is no detectable motion for that particular frame. This means that the overall scanning time is a function of the number of eye movement/blinking events during scanning. Unfortunately, as the number of eye movement/blinking events increases with age and severity of vision impairment, the overall scan time may become sometimes intolerably long for such cases if a dense 3-D volume scan is needed. This also indicates that the scanning speed of the current SD-OCT is still not fast enough to capture “motion-free” 3-D OCT image.
Swept-source OCT
Swept-source (SS) OCT is similar to SD-OCT in the use of detected wavelength to determine reflection depth. In SS-OCT, an A-scan line is obtained by scanning with a starting frequency and then “sweeping” the frequency over a period of time to obtain the different spectral components of the detected signal, using a tunable laser source with a continuous “sweeping” mode. Instead of using a spectrometer to determine different spectral components, which is the speed bottle neck in SD-OCT, a single detector gathers all the information of the “sweep” [7]. The acquisition speed of SS-OCT is dictated by the tuning speed of the laser light source. It reaches 100,000 to 400,000 A-scans in one second, substantially increasing the scanning speed to 4 to 8 times faster than SD-OCT. SS-OCT has been reported to scan at MHz rates as well [8–10].
With the dramatically improved scanning speed, regular 200 × 200 A-scans covering a 6 × 6 mm area can be done within a fraction of a second, and therefore we are getting one step closer to motion-free 3-D OCT images even without an eye-tracking system. However, the faster speed is generally used for denser sampling so that the overall scanning time for a 3-D cube scan remains about one second. This is because the sampling density has not reached the optimal number for both qualitative and quantitative assessment yet. Even with potential eye movement artifacts, denser sampling gets higher priority in OCT imaging for both clinical and research purposes.
Tunable laser light sources dramatically improve the scanning speed; however, they also bring disadvantages. The major disadvantage is the lower axial resolution than SD-OCT (5.3 to 8 μm (SS-OCT) vs. 4 to 5 μm (SD-OCT)). [11] Ironically, the narrow bandwidth of the swept-source laser, which achieves faster scanning, decreases the axial resolution because resolution is proportional to the bandwidth. Therefore, a narrower-bandwidth light source results in worse axial resolution
On the other hand, SS-OCT does not exhibit some of the problems seen with SD-OCT. Unlike with SD-OCT, neither the sensitivity nor the resolution of SS-OCT diminishes with increasing distance from the “zero-delay.” The zero-delay can be thought of as the point in space representing the beginning of each given A-scan, such that the farther a point is from the zero-delay, the deeper into the tissue the scan point is acquired.
Adaptive optics optical coherence tomography
OCT technology is known to provide great axial resolution (4 to 5 μm with SD-OCT). However, the transverse, or lateral, resolution is still limited to the range of 15 to 20 μm. This asymmetric proportion of the resolution in different dimensions hinders its visualization clarity in the coronal plane. For example, inner and outer borders of the photoreceptor layer together with the inner/outer segment junction can be recognized in axial slices, while the photoreceptor mosaic cannot ordinarily be visualized except in high A-scan density coronal slices. [12,13]
Adaptive optics (AO) was originally developed and used in astronomical telescopes in order to improve the resolution of astronomical imaging and remove the effects of atmospheric distortion. [14] It is now incorporated into OCT devices to correct ocular aberrations. AO systems measure the distortion in the wave shape of the reflected signal and compensate for them with a deformable mirror. In this way, AO fixes the ocular aberrations caused by wavefront distortions when the signal passes through the eye and, therefore, is able to markedly improve transverse resolution. Combined with OCT, it provides detailed visualization of individual retinal cells and generates 3-D images of photoreceptors in vivo. Since AO-OCT has better focus on the retina and more efficient collection of backscattered light by reducing the diffraction effect, it enhances image contrast and quality as well as transverse resolution. With a pupil diameter of > 6 mm, a transverse resolution of 2–3 μm can be achieved, which enables visualization of individual rods and cones as well as individual nerve fiber bundles [15,16].
Doppler OCT
Assessment of retinal vasculature and blood flow is an important clinical observation for evaluating various pathological conditions and understanding pathophysiology. Conventionally, fluorescein angiography (FA) and indocyanine green angiography (ICGA) have been used to observe the retinal blood vessel morphology, and Doppler ultrasound has been used for blood flow measurement. However, FA and ICGA imaging are invasive due to the requirement of dye injection [17, 18], and Doppler ultrasound can target only one or two vessels at a time and its measurement is largely operator dependent.
Doppler OCT (D-OCT) was developed for observation of both the vasculature morphology and blood flow measurement in a noninvasive fashion. The principle behind D-OCT is similar to the Doppler ultrasound. It is based on the frequency shift caused by the moving blood cells. When the incident light signal is scattered or reflected back from moving particles, a shift in frequency will be observed due to the Doppler effect. The shift in the central frequency of the reflected light can be detected as the phase change between sequential A-scans [19].
With the ability to detect the frequency shifts or phase changes, D-OCT allows imaging blood vessels in vivo with high resolution and sensitivity and offers valuable information regarding the blood flow direction and velocity, as well as detailed structural investigation of the vasculature [20–28]. With sophisticated image-processing techniques, quantitative flow investigation of retinal perfusion is now possible with commercially available OCT devices, though it still remains challenging. The most important contribution of D-OCT is to provide 3-D tissue retinal microvascular information in a noncontact and noninvasive fashion compared to FA and ICGA; however, D-OCT cannot identify leakage of fluid from vessels. D-OCT uses Doppler to generate image contrast without the use of dye through a technique called optical coherence angiography (OCA) and, therefore, enables segmentation and display of vasculature. Enabling the 3-D visualization of the vasculature, especially retinal and choroidal vasculature, provides an alternative means for diagnosis and management of many retinal pathologies related to vasculature damage [29, 30].
Polarization-sensitive OCT
Conventional OCT visualizes different tissues (mainly various retinal layers) based on the differences in their optical indices of refraction. However, the intensity-based contrasts can be less optimal for distinguishing certain adjacent tissues (e.g., boundary between ganglion cell and inner plexiform layers), especially under pathological conditions. There are other types of optical properties that the conventional OCT is not detecting, such as absorption, fluorescence, luminescence, and polarization. Polarization sensitive (PS) OCT can assess birefringence and depolarization of target tissues with depth information.
Fibrous tissues (e.g., nerve fiber bundles) that consist of long parallel fibrils surrounded by tissues with different refractive indices exhibit birefringence. Multiple scattering at large particles or scattering at nonspherical particles causes depolarization of incoming light. PS-OCT visualizes tissue specific contrast by detecting these optical properties. Initially, PS-OCT was only implemented in TD-OCT but now is adapted to SD-OCT and able to provide a contrast-enhanced OCT image with better sensitivity in real time [31–35].
Retinal tissues show both good birefringence and depolarization effects, so they can be good targets for PS-OCT. Scanning laser polarimetry (SLP or GDx) has been used for glaucoma assessment by measuring the retardation amount of the laser light (RNFL birefringence) that is supposed to linearly correlate with RNFL thickness [36–41]. Several studies suggest that RNFL birefringence changes may precede RNFL thickness changes that can be measured using conventional OCT [42–45]. The biggest limitation of SLP is that it measures tissue retardation as a whole. In other words, the RNFL measurements are confounded by other birefringent tissues in the eye (e.g., cornea, vitreous, sclera, etc.).
Since PS-OCT allows a depth-resolved optical probe of birefringence, RNFL birefringence can be separately measured from other confounding structures with birefringence properties. RPE exhibits depolarization, so it can also be a good target for PS-OCT, especially in eyes with pathologies involving RPE (e.g., macular degeneration, pigment epithelial detachments, pseudo vitelliform dystrophy, etc.) [46]. With potentially more accurate and detailed assessment of birefringent tissues using PS-OCT, we may be able to detect minute changes in the integrity of nerve fiber bundles at much earlier stages than currently possible.
High penetration OCT
One of the limitations of conventional OCT is its relatively short penetration depth. Although the scanning window covers ~2mm in the axial direction, OCT signals quickly become attenuated below the RPE. In the ONH area, visibility of lamina cribrosa depends on the thickness of the pre-lamina tissue. Additionally, SD-OCT exhibits gradual image dynamic range loss along the depth. In other words, images look sharp on the top but blurry on the bottom. This is an inherent problem stemming from the SD-OCT principle. In cases in which the region of interest is a deep tissue (e.g., choroid, lamina cribrosa), the so-called enhanced depth imaging (EDI) technique can be applied, in which the dynamic range deterioration can be reversed (blurry top and sharp bottom) [47–63]. However, there are other ways to overcome this limitation, such as by using a longer wavelength light source (so-called high penetration (HP) OCT).
Conventional OCT devices use infrared light with center wavelengths around 830 nm as the light source because signals around 830 nm are widely available and are well transmitted by water, the main content of the vitreous [64]. However, signals with wavelengths of 830 nm have limited reflected signal strength beyond the RPE and, therefore, limit the ability to visualize the deeper structures. Instead of using 830 nm, HP-OCT seeks an alternative local minimum on the spectrum of the water absorption, which is located around 1,050 nm, and uses it as the light source. Besides minimal absorption by the water, signals with wavelength around 1,050 nm exhibit less scattering in the choroid and less attenuation in the RPE. Therefore, signals can penetrate beyond the RPE and enable the visualization of tissue structure of choroid and sclera with better image quality and contrast [65–67]. This approach is different from EDI in using a longer wavelength light source.
HP-OCT has been employed in several studies to evaluate the high-penetration effects and clinical benefits [67–76]. HP-OCT is able to provide information posterior to the retina, like choroid, choroidal vasculature, and sclera, to observe choroid or sub-RPE damage or morphological changes caused by various pathologies, such as the development of choroidal neovascularization (CNV) beneath the RPE induced by age-related macular degeneration (AMD) and polypoidal choroidal vasculopathy (PCV) [67, 72], and to evaluate choroidal thickness with better reproducibility. Some statistically significant differences in choroidal thickness or morphological changes of the ocular tissues between healthy and pathological cases have been reported [73, 75, 76]. Furthermore, combining the OCA technology with an HP-OCT engine, high-penetration optical coherence angiography (HP-OCA), enables vascular imaging of the deep posterior eye in a noninvasive manner. A recent study showed that HP-OCA revealed depth-resolved abnormal vasculature in exudative macular disease and the en face HP-OCA images showed high similarity with FA and ICGA images. This suggests that HP-OCA may be clinically useful in noninvasive 3-D angiography [74].
Joint aperture OCT
As we have seen so far, improving the scanning speed has been one major focus of OCT technology advancements. However, it is known that higher speeds may degrade image quality due to shorter exposure time. One of the key components that dictate overall image quality is sensitivity, the minimum detectable fraction of back reflected light. Naturally, under the same illumination condition (light source power in OCT domain), shorter exposure lowers sensitivity. One way to overcome this law of physics is a new approach called joint aperture (JA) OCT.
JA-OCT is an angle-resolved OCT method, in which illumination from an active channel is simultaneously probed by several passive channels. In other words, JA-OCT combines multiple imaging channels that are targeting the same subject from different angles that are strategically placed. JA-OCT increases the collection efficiency and effective sensitivity of the OCT system without increasing the light source power on the sample, hence no further exposure or safety risk. Additionally, JA-OCT provides angular scattering information about the sample in a single acquisition, so the OCT imaging speed is not reduced. Therefore, JA-OCT is especially suitable for ultrahigh-speed in vivo imaging [77]. Based on the angle diversity concept, JA-OCT is able to generate a high-quality image with reduced speckle noise without sacrificing imaging speed by organizing multiple channels in an asymmetric configuration and varying beam sizes. With multiple channels, JA-OCT provides angular scattering information about the sample in a single acquisition, which may be useful for automatic tissue identification detection [78].
Klein et al. demonstrated that, scanning with JA-OCT, the acquisition time for human retina with 2,112 × 258 axial scans for each channel is 0.46 seconds [77]. The acquired data showed significantly reduced speckle noise, increased collection efficiency, higher signal-to-noise ratio (SNR), reduction of specular and parasitic reflections, and better visualization of retinal layers. With all these advantages, JA-OCT successfully compensates the dim signal level problem of ultrahigh-speed OCT devices, and provides high-quality images with substantially reduced speckle noise at megahertz imaging speed. JA-OCT may be able to provide more ocular tissue structural information, originally hidden because of limited image acquisition speed and image quality. Furthermore, JA-OCT also offers new perspectives for OCT functional imaging: increased channels may allow for reconstruction of the true Doppler vector and the angular scattering characteristics may make tissue identification feasible.
Conclusions
OCT is a rapidly evolving technology. The advances described in this section represent only a small number of the many areas in which OCT is continuing to develop. As lasers improve and prices drop, other new OCT iterations will appear, such as is possible in laboratories today with vertical cavity lasers permitting OCT imaging of the entire length of the eye in a single A-scan. The excitement and possibilities in OCT development are palpable.
References
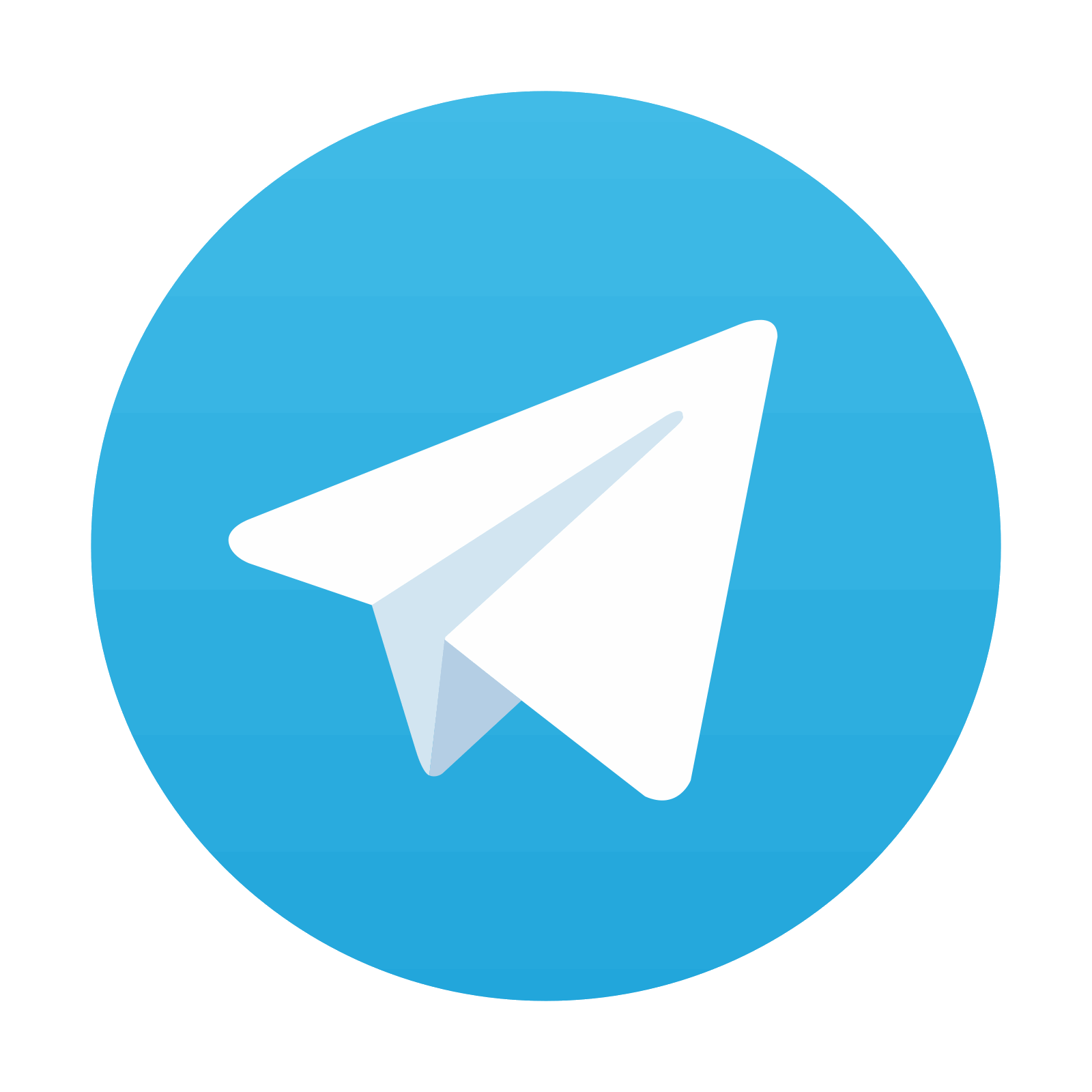
Stay updated, free articles. Join our Telegram channel
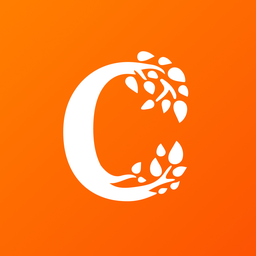
Full access? Get Clinical Tree
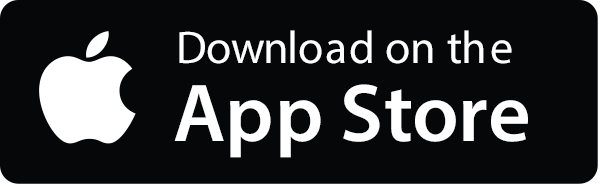
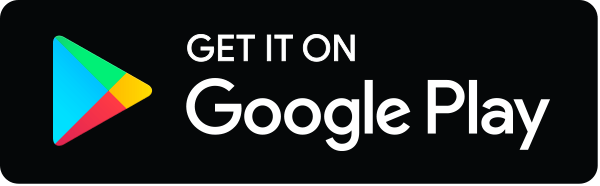