Low-contrast letter acuity chart (low-contrast Sloan letter chart, Precision Vision, LaSalle, IL).
* These charts have a standardized format based on the Early Treatment Diabetic Retinopathy Study visual acuity charts, the standard used in ophthalmology clinical trials, and they have several advantages over standard Snellen charts or near vision testing cards as traditionally used in MS trials: (1) letters (Sloan letters) are designed to be equally detectable for normal observers; (2) each line has an equal number of letters (five per line); (3) spacing between letters and lines is proportional to the letter size; (4) the change in visual acuity from one line to another occurs in equal logarithmic steps (a change of three lines constitutes a doubling of the visual angle); (5) visual acuity (for high-contrast [black letters on white] chart) may be specified by Snellen notation for descriptive purposes (i.e., 20/20), by the number of letters identified correctly. This figure shows the 25% contrast level for purposes of illustrating the format; the actual contrast levels used in these trials, 2.5% and 1.25%, have substantially lighter gray letters. The charts measure 14 × 14 inches for easy use and portability in the MS clinical trial setting; charts may also be mounted on a retroilluminated cabinet, thus, eliminating the need for standardization of room lighting levels.
Table 6.1 presents data from studies that have examined LCSLC using the Sloan charts, as well as high-contrast VA by ETDRS, OCT measures, and quality-of-life (QOL) scales using MS, optic neuritis (ON), and disease-free controls as an example [1]. These data may be used to provide initial reference values and should be viewed in the context of (1) the continually evolving field of vision in MS and neurologic disease, (2) the potential challenge inherent in applying group data to individual patients, and (3) the perspective that even the small observed differences in mean OCT values have been shown to correlate with clinically meaningful changes in visual function and QOL.
Disease-Free Controls | All MS | MS, No History of ON | MS, History of ON | References for Data * | |
---|---|---|---|---|---|
High-contrast visual acuity (VA), ETDRS, number of letters correct | 64 ± 5 | 59 ± 8 | 60 ± 6 | 58 ± 9 | 173 * |
(n=61 eyes) | (n=239 eyes) | (n=150 eyes) | (n=87 eyes) | ||
Binocular testing | 66 ± 5 | 62 ± 8 | 63 ± 7 | 61 ± 10 | 62 * |
(n=324 pts) | (n=1,007 pts) | (n=544 pts) | (n=463 pts) | ||
Low-contrast letter acuity (2.5%), number of letters correct | 34 ± 8 | 26 ± 11 | 28 ± 9 | 22 ± 12 | 173 * |
(n=61 eyes) | (n=239 eyes) | (n=150 eyes) | (n=87 eyes) | ||
Binocular testing | 43 ± 6 | 36 ± 10 | 38 ± 9 | 35 ± 11 | 62 * |
(n=324 pts) | (n=1,007 pts) | (n=544 pts) | (n=463 pts) | ||
Low-contrast letter acuity (1.25%), number of letters correct | 25 ± 7 | 16 ± 10 | 18 ± 10 | 11 ± 11 | 173 * |
(n=61 eyes) | (n=239 eyes) | (n=150 eyes) | (n=87 eyes) | ||
Binocular testing | 34 ± 8 | 24 ± 11 | 26 ± 11 | 22 ± 12 | 62 * |
(n=324 pts) | (n=1,007 pts) | (n=544 pts) | (n=463 pts) | ||
NEI-VFQ-25 composite score, | |||||
best score = 100 | 96 ± 4 (n=31 pts) | 88 ± 13 (n=122 pts) | 90 ± 12 (n=111 pts) | 85 ± 14 (n=51 pts) | 173 * |
10-Item Neuro-Ophthalmic | |||||
Supplement to the NEI-VFQ-25, | 97 ± 3 | 87 ± 13 | 88 ± 12 | 83 ± 14 | 173 * |
best score=100 | (n=31 pts) | (n=122 pts) | (n=111 pts) | (n=51 pts) | |
Time-domain (TD) OCT | |||||
Peripapillary RNFL thickness, μm | 104.5 ± 10.7 | 92.5 ± 16.7 | 95.6 ± 14.5 | 85.7 ± 19.0 | 174 * |
(n=219 eyes) | (n=1,058 eyes) | (n=730 eyes) | (n=328 eyes) | ||
Total macular volume, mm3 | 6.84 ± 0.36 | 6.54 ± 0.51 | 6.63 ± 0.48 | 6.36 ± 0.53 | 174 * |
(n=219 eyes) | (n=1,058 eyes) | (n=730 eyes) | (n=328 eyes) | ||
Spectral-domain (SD) OCT | |||||
Peripapillary RNFL thickness, μm | 92.9 ± 10.0 | 84.3 ± 12.8 | 87.6 ± 11.1 | 78.4 ± 13.6 | 173 * |
(n=61 eyes) | (n=239 eyes) | (n=150 eyes) | (n=87 eyes) | ||
Ganglion cell + inner plexiform layer (GCL+IPL), μm | 88.9 ± 6.9 (n=61 eyes) | 84.1 ± 8.4 (n=239 eyes) | 87.0 ± 6.6 (n=150 eyes) | 79.7 ± 9.2 (n=87 eyes) | 173 * |
Macular RNFL, μm | 29.6 ± 6.0 | 23.5 ± 8.2 | 25.5 ± 7.1 | 20.0 ± 9.0 | 173 * |
(n=61 eyes) | (n=239 eyes) | (n=150 eyes) | (n=87 eyes) |
Abbreviations: MS = multiple sclerosis; ETDRS = Early Treatment Diabetic Retinopathy Study; QOL = quality of life; NEI-VFQ-25 = 25-Item National Eye Institute Visual Functioning Questionnaire; TD = time-domain (OCT-3 platform); SD = spectral-domain (Cirrus platform); OCT = optical coherence tomography; RNFL = retinal nerve fiber layer
* Reference with asterisk is source of data presented in table; other published studies contain similar data. Table adapted from reference [1].
Low-contrast letter acuity: clinical correlates
On a standard Snellen VA chart, a 20/20 letter occupies a very small area (0.083 degree of vision), which is equivalent to 18 to 24 cycles per degree, the high-frequency end of the contrast-sensitivity function [3]. On the other hand, the peak of contrast sensitivity occurs between a spatial frequency of 3 and 6 cycles per degree (the equivalent visual frequency of the 20/200 line on the Snellen chart), a range responsible for activities like discerning a bus from a car [3]. This peak contrast sensitivity has been shown to be the most important visual factor in predicting performance on activities of daily function [3].
Far from being an academic exercise without any real-world applications, low-contrast vision has been shown to play an important role in the ability to carry out activities of daily living (e.g., pouring liquids, using tools) [46, 47], reading [31, 47–49], mobility [50], negotiating stairs [51], driving [52], and facial recognition [53–55], and influences perceived disability [56]. Further, diminished contrast sensitivity has been associated with an increased risk for falls in older adults [57, 58].
There is evidence that impaired contrast sensitivity is as disabling as visual field loss and more disabling than VA loss [59]. Decreased contrast sensitivity has been shown to negatively impact patients’ quality of life. In a study of multiple sclerosis (MS) patients, a two-line difference in low-contrast acuity was associated with a deterioration of greater than four points on the NEI-VFQ-25 (25-Item National Eye Institute Visual Functioning Questionnaire) composite score, indicating an impaired quality of life [60]. The NEI-VFQ-25 domains most associated with diminished contrast acuity in this study included those for general vision, near and distance activities, role difficulties, and driving. Reduced contrast sensitivity was also associated with poorer scores on other measures of quality of life, including the Impact of Visual Impairment Scale (IVIS) and the Short Form 36 Health Survey (SF-36) [60]. Decreased binocular low-contrast vision has also been shown to correlate with the Extended Disability Status Scale (EDSS) and the MS Functional Composite (MSFC) scores, both measures of disability in MS patients [39]. In fact, deterioration of low-contrast vision can predict future EDSS decline [39].
How well a patient sees in high-spatial-frequency channels, which is captured by standard tests of high-contrast visual acuity (e.g., Snellen charts), does not necessarily predict visual function at intermediate or lower frequencies [2, 3, 61]. Contrast sensitivity testing can detect apparently occult evidence of visual dysfunction that would otherwise be absent on standard tests of visual acuity. As will be discussed later, a number of disorders can often affect contrast sensitivity and spare high-contrast visual acuity, especially early in the course of the disease. Thus, contrast sensitivity is a sensitive measure of disease progression, and it is a valuable clinical trial outcome tool in ophthalmic and neurologic disorders.
Binocular summation and binocular inhibition
VA may be measured monocularly (with either eye separately) or binocularly (with both eyes together). While measuring binocular acuity saves time and avoids patient fatigue, comparing monocular to binocular scores is important to ascertain the effects of binocular summation or inhibition [62]. Binocular summation is a phenomenon in which VA improves under binocular viewing conditions (i.e., better than the scores of either eye). In contradistinction, binocular inhibition results in worse VA than when viewing with either eye alone [62, 63]. Low-contrast acuity has been shown to be more sensitive than high-contrast VA in assessing the effect of binocular summation [62, 64].
Two hypotheses have been proposed to explain the phenomenon of binocular summation. “Probability Summation” assumes complete independence of the two eyes and predicts enhancement of binocular over monocular vision due to the statistical consideration that a binocular observer has two opportunities to detect weak signals. “Neural Summation” posits that binocular superiority exceeds that which would be expected from probability summation alone and attributes this phenomenon to cortical interactions (layer VI) [65]. The deterioration in binocular summation following acute optic neuritis (AON) may be a consequence of complex cortical reorganization and support the neural summation hypotheses [66]. Decreased binocular summation, or frank binocular inhibition, in MS patients is more common in older age groups, those with a prior history of AON, and those with a greater inter-ocular VA difference [62]. Better binocular summation was associated with higher NEI-VFQ-25 scores (which, in turn, suggests a better quality of life) [62]. Alternately, binocular summation is decreased with age [64] and in strabismus [65].
Disorders affecting low-contrast vision
Contrast sensitivity can be affected as part of the normal aging process, as well as in various ophthalmic, neurologic, and psychiatric conditions. Impaired contrast sensitivity has been observed in myopia [67], cataract [68, 69], pseudophakia [70], meridional ambylopia [71], strabismus [65], dermatochalasis [72], nonpenetrating corneal foreign bodies [73], age-related macular degeneration [74–76], xerophthalmia [77], birdshot chorioretinopathy [78], retinitis pigmentosa [79–80], diabetic retinopathy [81, 82], and glaucoma [83–85].
In addition to MS, other neurologic disorders that may compromise low-contrast vision include Parkinson’s disease [38, 86–89], Alzheimer’s disease [20, 90–92], Duchenne muscular dystrophy [93], neurofibromatosis type 1 [94], migraine [95, 96], pseudotumor cerebri [97–100], Friedreich’s ataxia [101], a left parieto-occipital infectious lesion, and a left occipital meningioma [102]. Reduced contrast sensitivity has also been observed in schizophrenia [103] and depression [104, 105]. Diminished contrast sensitivity is a common, albeit under recognized, side effect of many medications, including ethambutol [106] and tiagabine [107]. Exposure to solvents has also been implicated in decreased contrast sensitivity [108–110].
Impaired contrast sensitivity under photopic conditions with increasing spatial frequencies occurs in older adults, as part of the normal aging process, due to both optical and neural changes [111, 112]. By age 75, almost half of adults have cataracts, which impair contrast sensitivity by absorbing light, scattering light, and increasing disability glare [69, 113]. Notwithstanding the effects of pupillary miosis, higher lens density, increased optical aberrations, and increased intraocular light scattering on retinal illuminance, age-related visual pathway changes also conspire to further diminish contrast-sensitivity in older adults [64, 111]. Rod photoreceptor density is particularly affected by aging compared to cone photoreceptor density, which remains relatively stable [114]. Rod photoreceptor degeneration may result from deficits in rhodopsin regeneration [113]. Furthermore, at the cortical level, studies in senescent monkeys have demonstrated that cortical neurons exhibit lower optimal spatial and temporal frequencies, decreased contrast sensitivity, diminished spatial resolution, and reduced higher temporal frequency cut-offs when compared to younger monkeys [115, 116]. FDT perimetry has been shown to be useful in detecting age-related contrast-sensitivity impairment [112, 117, 118].
The progressive loss of dopaminergic neurons, the hallmark of Parkinson’s disease (PD), also affects the retina [119]. Retinal dopaminergic deficiency is hypothesized to cause physiological dysfunction of dopaminergic amacrine, horizontal, and interplexiform cells that subsequently compromises the receptive field properties of RGCs. Pattern electroretinography (PERG) can be used to detect RGC dopaminergic dysfunction in PD patients, and, indeed, levdopa treatment improves delayed PERG latencies [89, 113, 120, 121]. Compared to the standard, high-contrast checkerboard pattern, low-contrast PERG is more sensitive in detecting RGC dysfunction in PD [88, 89]. Likewise, contrast sensitivity using chart-based testing is diminished in PD [38, 87]. Besides retinal abnormalities, pathologic changes elsewhere in the afferent visual pathway contribute to visual dysfunction in PD patients. Specifically, the lateral geniculate nuclei and visual cortices may also be affected by the neurodegenerative processes of PD [122–124]. Orientation-specific loss of contrast sensitivity in PD may provide evidence for cortical involvement [125, 126].
Several studies have reported impaired contrast sensitivity in Alzheimer’s disease (AD) [90–92]. Using FDT, a more recent study [20] showed that decreased contrast sensitivity, most notably in the bilateral upper-right visual field quadrant, was associated with poorer cognitive measures in AD and mild cognitive impairment. In AD, the outer retina (i.e., photoreceptors) is normal, as evidenced by normal full-field electroretinography [127]. However, like PD, the PERG is abnormal, signifying RGC dysfunction [127, 128], the possible consequence of retinal amyloid deposition [129]. Specifically, electroretinographic abnormalities are most pronounced for high-temporal-frequency patterns, indicating selective impairment of the large M-type RGCs [128]. Pathologically, neurodegenerative changes have been noted in the central M-pathway in the lateral geniculate nucleus and visual cortices [130–134], as well as in the retina [135–142]. Furthermore, glaucoma, cataracts, and macular degeneration (all of which also compromise contrast sensitivity) are more frequent among AD patients [143].
Structure meets function: low-contrast acuity and OCT in multiple sclerosis
MS is arguably the neurologic disorder in which low-contrast acuity, as well as OCT, have been most widely tested in recent years. MS frequently affects both the afferent and efferent visual pathways. Visual dysfunction occurs in 80% of patients and is the presenting feature in 50% [144, 145]. Acute optic neuritis (AON) is the presenting feature in 20% of cases, and 50% of MS patients develop AON during the course of their disease [146]. However, virtually all patients have evidence of optic nerve demyelination at autopsy [147, 148], indicating that occult, subclinical optic nerve demyelination occurs in the vast majority of MS patients, whether or not they develop clinical symptoms and signs of AON.
The eloquence of the afferent visual pathway and the frequency of AON in MS make it an ideal system to use as a model to understand the pathobiological underpinnings of the disease, as well as to develop sensitive biomarkers that can be applied to clinical trials of neuroprotective, and even neuro-restorative, therapies. The robust utility of optical coherence tomography (OCT) in detecting axonal damage in MS is explored at length throughout this book, but it remains possible that early pathologic changes that begin at a cellular level (particularly in the postgeniculate visual pathway) may easily escape the resolution of even the most sensitive OCT. Before any structural changes become evident, these pathologic perturbations may manifest as functional abnormalities that may be detected by means of sensitive electrophysiological assessments or tests of visual function. It is important to identify such early dysfunction, since it may represent a potentially reversible state of visual compromise; detecting these abnormalities would permit timely therapeutic interventions that would potentially stop the disease process, prevent future disability, and even reverse the damage.
Although the EDSS [149] is widely used as an outcome measure for clinical trials in MS, this scale is heavily skewed in favor of the motor system involved in ambulation and lacks any sensitivity with regard to other salient manifestations of MS-related disability, including fatigue, heat sensitivity, cognition, and, in particular, visual function. The MSFC was developed to serve as a more sensitive measure of the multidimensional manifestations of MS-related disability and included the quantitative tests of arm function (the 9-hole Peg Test), leg function (the timed 25-foot walk), and cognition (the PASAT3) [150–152], but it is devoid of any measure of visual function. For a long time, the only measure of visual function in MS patients was the hand-held Snellen chart, which measured high-contrast visual acuity (VA) [153].
While convenient and reproducible, the use of the Snellen chart in MS patients is limited by several inherent problems. First, Snellen VA is tested at the near distance, and it may potentially be difficult for presbyopic patients [32]. Second, despite normal high-contrast VA, there may be pathologic evidence of severe visual pathway demyelination [147] and RNFL thinning [154, 155]. Finally, as discussed earlier, Snellen VA only tests higher-spatial-frequency levels and is insensitive to impairment of intermediate to lower spatial frequencies. By contrast (pun intended), low-contrast vision testing has proven to be a more sensitive measure of visual function in MS. Many studies have shown that, despite recovery of standard, high-contrast VA to 20/20 or better following an attack of AON, patients continue to have persistent abnormalities of low-contrast vision [32, 34–37, 43, 44, 153–171].
Decreased contrast acuity correlates with RNFL thinning and reduced macular volume [44, 154, 155]. Poorer contrast sensitivity has been associated with a higher MS lesion load, particularly in the postgeniculate visual pathway [168, 172], suggesting that postgeniculate visual processing plays an important part in contrast sensitivity. It would be easy to assume that RNFL thinning reflects pregeniculate damage while impaired contrast sensitivity (without OCT changes) indicates postgeniculate lesions. Reich et al. [172], however, dispelled this simple dichotomy and showed that postgeniculate MS lesions were associated with RNFL thinning as well. The precise mechanisms for retinal changes resulting from postgeniculate damage remain unclear, but they may be related to trans-synaptic degeneration.
Further, low-contrast acuity been shown to be the best measure that distinguishes MS patients from disease-free controls, accounting for age [39, 153]. As a trial outcome for natalizumab (in the AFFIRM and SENTINEL clinical trials), low-contrast acuity was sensitive enough to detect treatment effects and visual changes sustained over time, demonstrating the superiority of natalizumab as a disease-modifying agent [43]. As discussed previously, contrast sensitivity correlates well with various measures of visual dysfunction and disability in MS.
Summary
While impaired low-contrast vision is not pathognomonic for any particular disease, the presence of diminished contrast sensitivity can alert the clinician to the presence of a pathologic disorder and help explain the bases of a patient’s visual complaints, particularly if other tests of visual structure and function are apparently normal. When used in conjunction with OCT, contrast sensitivity can provide useful and valuable information that will allow clinicians and researchers to follow disease progression. Contrast sensitivity testing can provide information that complements that garnered from structural (e.g., OCT) and functional measures (e.g., visual fields, color vision, electrophysiologic tests) of the afferent visual system. Contrast sensitivity can now be easily tested in busy clinical or research settings with commercially-available charts as well as established, efficient testing protocols. Evidence from MS clinical trials has demonstrated that contrast acuity is a reliable, sensitive measure of visual function that can be used to follow disease evolution and response to therapy.
References
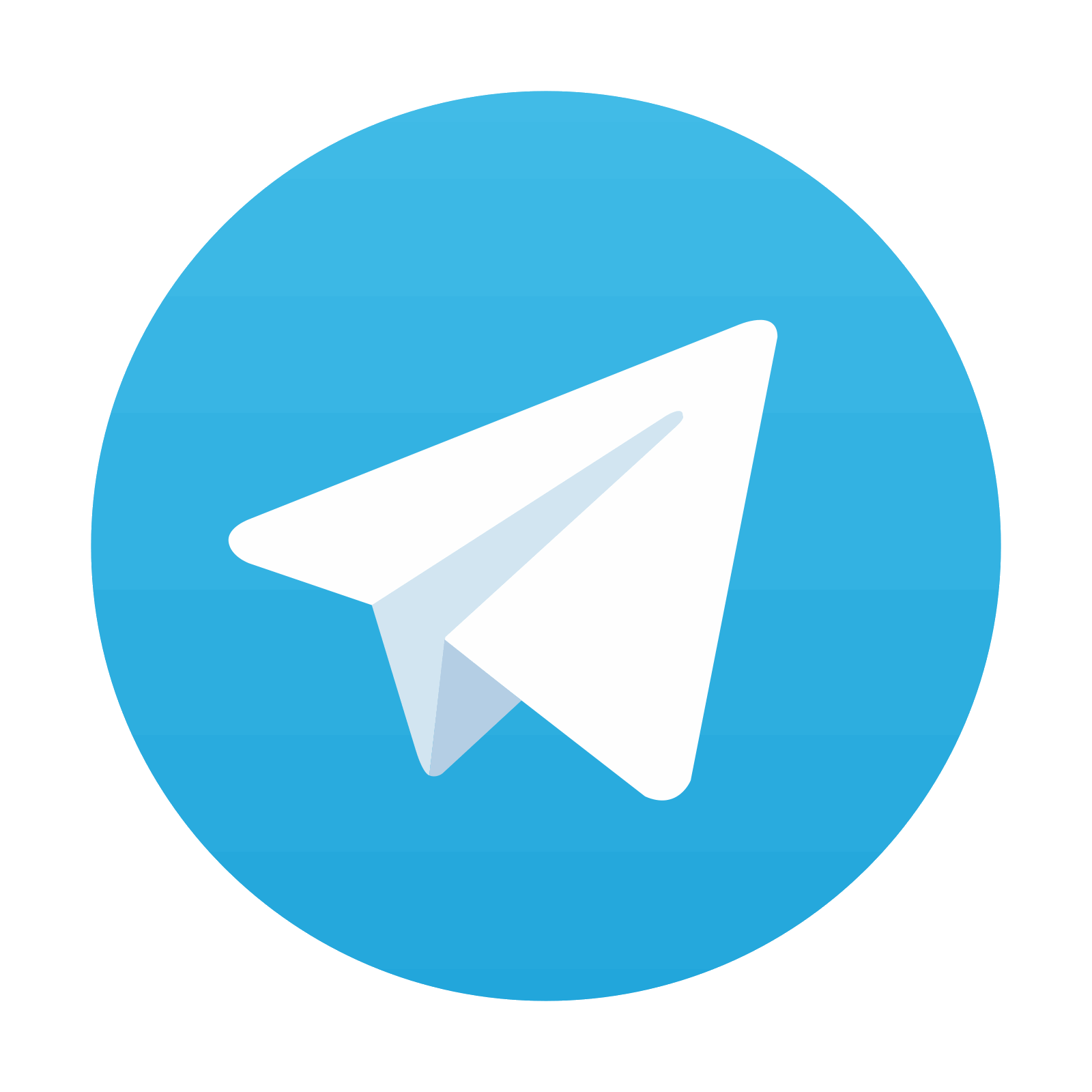
Stay updated, free articles. Join our Telegram channel
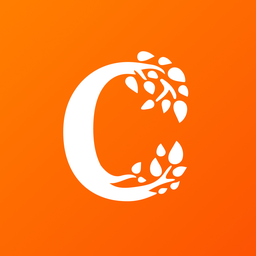
Full access? Get Clinical Tree
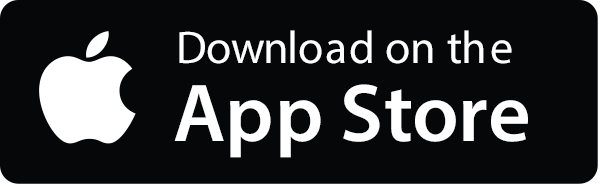
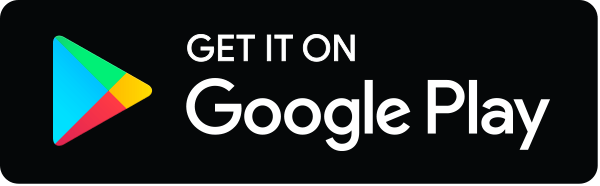