Technical Aspects of Transcranial Magnetic and Electrical Stimulation of the Brain
Alan D. Legatt
Alvaro Pascual-Leone
Alexander Rotenberg
Transcranial stimulation of the corticobulbar and corticospinal tracts within the brain is used for intraoperative monitoring and for extraoperative diagnostic assessment of central motor pathways. The brain may be stimulated using both transcranial electrical stimulation (TES) and transcranial magnetic stimulation (TMS). As will be shown, TES, especially TES utilizing brief trains of stimulus pulses, is more useful for intraoperative monitoring, while TMS is more useful for extraoperative diagnostic motor evoked potential (MEP) studies as well as research applications to establish and map causal brain-behavior relations in nonmotor cortical areas. TMS, especially repetitive stimulation using TMS, has also been used therapeutically, for example, for treatment of depression (1) and other psychiatric disorders (2), for improving motor function after strokes (3), and for other neurologic conditions. Both TES and TMS depolarize neurons by producing electrical current within brain parenchyma, although the ways in which these intraparenchymal currents are generated are different.
Applied to the motor cortex, a single electrical stimulus to the cerebral cortex may produce multiple volleys within the descending motor tracts. Studies in experimental animals have elucidated the mechanisms underlying this (4) (Fig. 52.1). The first volley reflects direct stimulation of the pyramidal neuron axons that leave cerebral cortex and comprise the corticobulbar and corticospinal tracts; this volley has been labeled the D-wave (“D” for “direct”). Subsequent volleys, labeled I-waves (“I” for “indirect”) derive from activation of the pyramidal neurons via excitatory synaptic input from other cortical neurons that were themselves activated, either directly or indirectly, by the externally applied stimulus. The delay from that stimulus to an I-wave reflects the time required for the intervening synaptic transmission(s), and thus is the sum of an integral number of cortical synaptic transmission delays. This accounts for the relatively consistent intervals between the D-wave and the first I-wave and between successive I-waves (e.g., see Fig. 52.1A). Since the production of I-waves depends on cortical synaptic transmission, factors that depress cortical synaptic function will reduce or eliminate the I-waves (Fig. 52.1B). Most anesthetic agents depress cortical synaptic function, and will reduce or eliminate the I-waves (5, 6 and 7) (Fig. 52.2).
In TES, stimulating current passes between an anode and a cathode that are at different locations on the scalp. With electrical stimulation of peripheral nerves, the action potential volleys are predominantly initiated under the cathode (8), whereas with TES the D-waves are predominantly initiated under the anode (4) (Fig. 52.3). Why is this? Outward transmembrane current
depolarizes the neuronal membrane and is excitatory, whereas inward transmembrane current hyperpolarizes the neuronal membrane and is inhibitory. When stimulating peripheral nerve, the outward transmembrane current depolarizes the axonal membrane and initiates the action potential under the cathode (Fig. 52.4). When stimulating cerebral cortex, it is the action potentials that are propagating in the corticofugal motor tracts that generate the MEPs, and these action potentials are initiated at the axon hillocks of the cortical pyramidal neurons. Current flows under the scalp anode result in inward (inhibitory) transmembrane current in the superficial portion of the pyramidal neuron but outward (excitatory) current at the axon hillock (9) (Fig. 52.5), which produces the D-waves. Current flows under the TES cathode are opposite in direction; they may excite the superficial portion of the pyramidal neuron as well as cortical interneurons, but the hyperpolarization at the axon hillock may block the initiation or propagation of action potentials along the motor tracts that would give rise to D-waves. Under surgical anesthesia, when I-waves are largely suppressed and the MEPs are predominantly generated by D-waves, the myogenic MEPs elicited by TES are predominantly recorded from muscles contralateral to the TES anode (Fig. 52.6).
depolarizes the neuronal membrane and is excitatory, whereas inward transmembrane current hyperpolarizes the neuronal membrane and is inhibitory. When stimulating peripheral nerve, the outward transmembrane current depolarizes the axonal membrane and initiates the action potential under the cathode (Fig. 52.4). When stimulating cerebral cortex, it is the action potentials that are propagating in the corticofugal motor tracts that generate the MEPs, and these action potentials are initiated at the axon hillocks of the cortical pyramidal neurons. Current flows under the scalp anode result in inward (inhibitory) transmembrane current in the superficial portion of the pyramidal neuron but outward (excitatory) current at the axon hillock (9) (Fig. 52.5), which produces the D-waves. Current flows under the TES cathode are opposite in direction; they may excite the superficial portion of the pyramidal neuron as well as cortical interneurons, but the hyperpolarization at the axon hillock may block the initiation or propagation of action potentials along the motor tracts that would give rise to D-waves. Under surgical anesthesia, when I-waves are largely suppressed and the MEPs are predominantly generated by D-waves, the myogenic MEPs elicited by TES are predominantly recorded from muscles contralateral to the TES anode (Fig. 52.6).
While I-waves are mediated by cortical synaptic activity, D-waves can be elicited by stimulation of corticospinal tract axons within the white matter (Fig. 52.1A,C). As the TES stimulus intensity is increased, intraparenchymal current densities capable of stimulating these axons are produced further and further away from the surface of the head, and may be able to stimulate them as far caudally as the medulla (10). During intraoperative monitoring of the brainstem and of the corticospinal tracts within the cerebrum, this could prevent recognition of surgery-related motor tract compromise if the motor tracts were stimulated caudal to that dysfunction.
TRANSCRANIAL MAGNETIC STIMULATION
During TMS, high-intensity current pulses are passed through a coil that is held in close proximity to the patient’s head. The magnetic field produced by this coil passes through the skull without significant attenuation and induces rotatory currents within the patient’s brain that flow in the opposite direction to the current in the stimulating coil (11) (Fig. 52.7). In essence, this system functions as a transformer, with the brain parenchyma acting as the secondary coil. It is the current induced by the time-varying magnetic field within the brain that stimulates the neurons. Thus, TMS is essentially electrical brain stimulation, although the delivery of electromagnetic energy to the head is via a rapidly changing magnetic field-pulse rather than by directly conducted currents. The advantage of TMS is that it does not activate scalp pain fibers as strongly as TES, and it is therefore useful for assessing central motor pathways in conscious subjects. TMS can also be applied to nonmotor regions in the brain convexity. Depth penetration is limited, as the magnetic field strength decreases with the square of the distance and is always maximal closer to the stimulation coil. Therefore, selective stimulation of deep brain structures is not possible.
Pyramidal tract axons are most effectively stimulated by radial currents, those flowing normal to the cortical surface (Fig. 52.5). Depending on the position and orientation of the coil, the intraparenchymal currents induced by TMS may be largely tangential in orientation (Fig. 52.7), preferentially stimulating horizontally coursing neurites within the cortical neuropil rather than the axon hillocks of pyramidal neurons (Fig. 52.8). Thus, TMS often produces predominantly I-waves rather than D-waves (12,13) (Fig. 52.9). Since the I-waves are suppressed by surgical anesthesia (Fig. 52.2), anesthesia may also markedly suppress the MEPs elicited by TMS. In addition, small changes in the position and orientation of the stimulating coil may produce dramatic changes in TMS-MEPs (14), and it is difficult to maintain the coil in the exact same position relative to the patient’s head during operations lasting several hours. The use of image-guided neuronavigation systems can overcome this limitation, but adds complexity to the operating room setup. In addition, the magnetic field-pulse induced by TMS can affect other equipment in the operating room, necessitating appropriate placement and shielding of equipment. Therefore, TES is the procedure of choice for eliciting MEPs for intraoperative monitoring (15).
With a circular coil, the intraparenchymal current density is relatively consistent within the torus of brain tissue underlying the coil (Fig. 52.7). In order to obtain more focal stimulation of cerebral cortex, a figure-eight coil or other noncircular coil geometries may be used, to obtain a focally higher current density within a more restricted volume (16, 17 and 18) (Fig. 52.10). During diagnostic TMS-MEP studies, maintenance of a mild degree of tension in the muscle group(s) from which the MEPs are being recorded can augment the MEPs and decrease their onset latencies, or cause previously absent MEPs to appear (11,19). This is most likely due to the voluntary contraction maintaining the spinal motor neurons in a partially depolarized state (19).
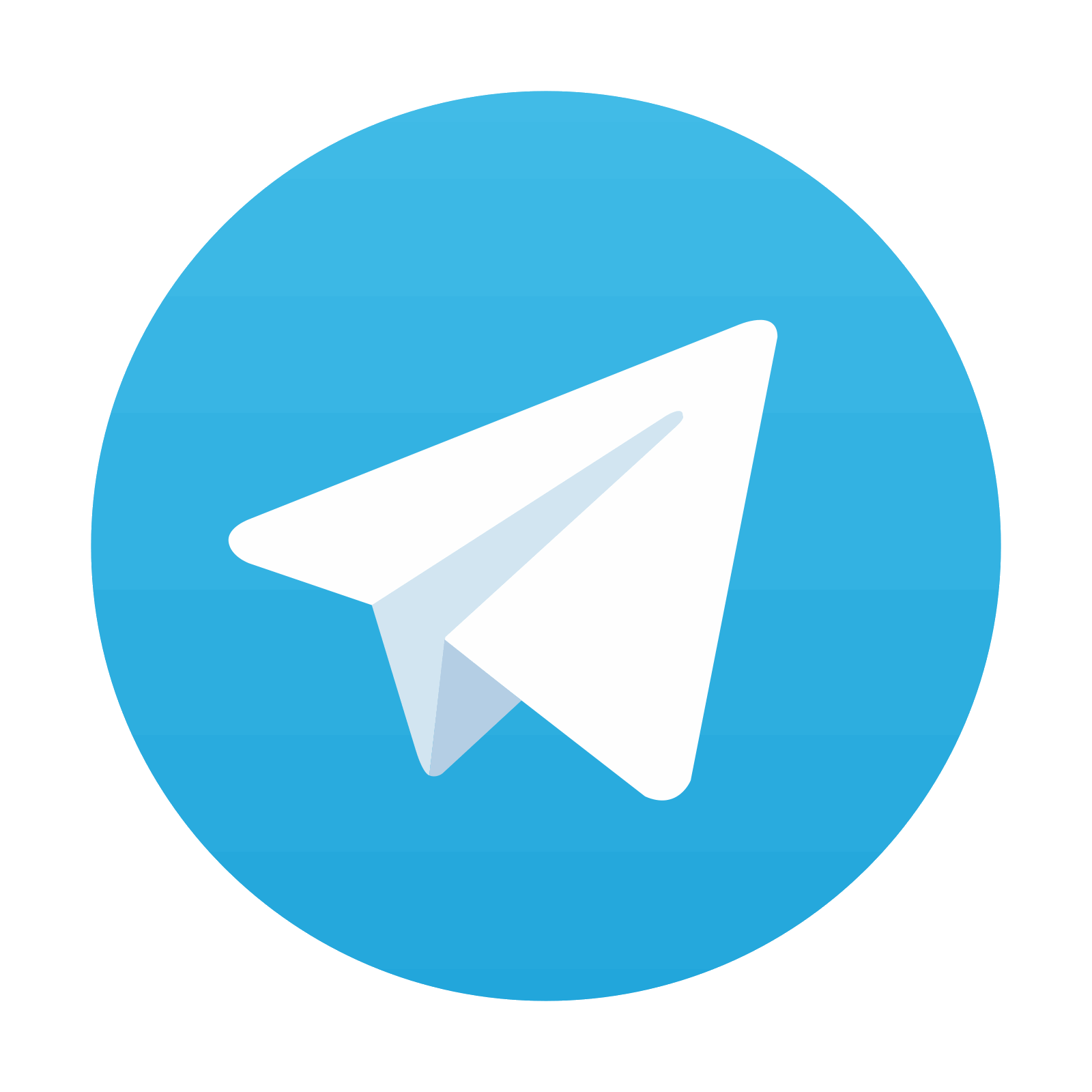
Stay updated, free articles. Join our Telegram channel
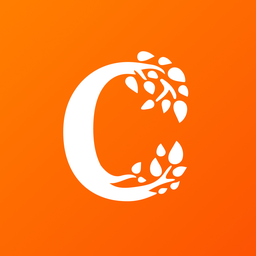
Full access? Get Clinical Tree
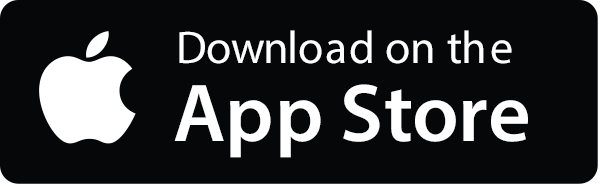
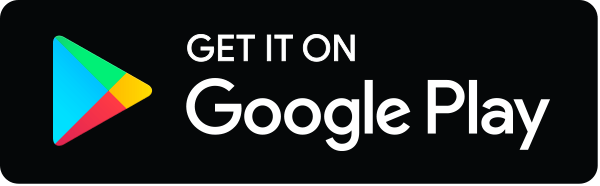