Fig. 3.1
Chemical structures of n-3, n-6, and monosaturated fatty acids
3.2 Transport and Incorporation of Fatty Acids in the Brain
In diet, saturated fatty acids, ARA, and DHA are present as triacylglycerols and phospholipids, which are hydrolyzed by lipases and phospholipases in gastric and intestinal lumen (Bezard et al. 1994). Fatty acids are incorporated in noticeable amounts in chylomicron phospholipids during the process of gastrointestinal absorption, and are also packaged into VLDL triglycerides by the liver. ARA and DHA can also be esterified into phospholipids and cholesterol esters associated with circulating lipopoproteins and only a small proportion of total plasma fatty acids is found in the FFA pool. Through the action of lipoprotein lipase bound to the luminal surface of endothelial cells, ARA and DHA are cleaved from circulating triglycerides where they can act as ligands or taken up by peripheral tissues (Polozova and Salem Jr. 2007).
Liver is one of the most active organs and its role is critical in providing long-chain polyunsaturated fatty acids (PUFA) secretion in VLDL (very low density lipoprotein) (Bezard et al. 1994). In liver, ARA and DHA associate themselves either to albumin and lipoproteins. From liver ARA and DHA are transported in the blood either bound to albumin or in the form of triacylglycerol associated with lipoproteins (Fig. 3.2). Understanding the mechanisms by fatty acids cross the blood brain barrier (BBB) and their utilization by neurons and glia is critical for understanding normal brain development and function. The rate of ARA and DHA crossing through BBB is higher from ARA and DHA-albumin complexes than from circulating ARA and DHA lipoproteins complexes (Hamilton and Brunaldi 2007; Moulle et al. 2012). The transport of ARA and DHA across BBB and other non-neural cellular membranes not only occurs through passive diffusion, but also through interaction with intracellular fatty acid binding proteins. These include membrane proteins fatty acid translocase (FAT/CD36), plasma membrane fatty acid-binding protein (FABPpm) and fatty acid-transport protein (FATP) (Utsunomiya et al. 1997). There are 10 members of FABP family. Three of them are associated with the development and function of the adult brain (Liu et al. 2010a, b), such as neurite formation, synapse maturation, neuron migration during brain development, and supports neuronal function in adult age (Sellner et al. 1995; Feng et al. 1994). Although the fine mechanism is still elusive, this is a fundamental process by which lipid-based molecules enter the cell for modulating signal transduction pathways along with activation of transcription factors. Adult mouse and rat brains abundantly express FABPpm and FATP (Greenwalt et al. 1995; Utsunomiya et al. 1997). These transporters provide a high-affinity mechanism for recruitment of ARA and DHA off the albumin. These binding proteins may also function in the fine tuning of cellular processes by modulating the metabolism of long-chain fatty acids implicated in the regulation of cell growth and various cellular functions (Dutta-Roy 2000; Glatz et al. 2001). In brain major proportion of ARA and DHA are activated and incorporated into neural membrane phospholipids through the action of Land cycle enzymes (acyl-CoA synthases and acyl-CoA: lysophospholipid acyltransferases) (Farooqui et al. 2000a). ARA and DHA are mainly located at the sn-2 position of glycerol moiety of neural membrane phospholipids. A small amount of eicosapentaenoic acid (EPA, 20:5n-3) is also present at the sn-2 position of glycerol moiety. Incorporation and enrichment of ARA and DHA in neural membranes not only provide them with stability, fluidity and permeability, but also contribute to the optimal function of integral membrane proteins, receptors, transporters, and ion-channels (Farooqui et al. 2000b; Horrocks and Farooqui 2004; Farooqui and Horrocks 2007). In addition, phospholipids act as a reservoir for phospholipid-derived lipid mediators, which are lipophilic molecules that facilitate signal transduction processes, regulate cell-cell communication, neural cell proliferation, and differentiation along with control of molecular and cellular processes involved in neuroinflammation , oxidative stress, and gene expression in the brain (Farooqui 2011) .
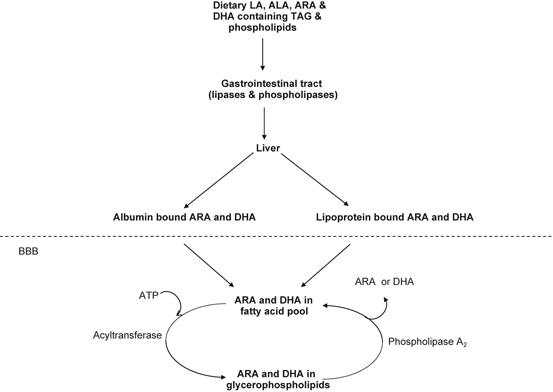
Fig. 3.2
Transport of LA, ALA, ARA, and DHA containing TAG and phospholipids in the brain. Linoleic acid (LA), α-linolenic acid (ALA), arachidonic acid (ARA), docosahexaenoic acid (DHA), and triacylglycerol (TAG)
3.3 Release of ARA and DHA from Neural Membrane Phospholipids
As stated above, proportions of ARA and DHA in neural membrane phospholipids vary considerably in the various subclasses of phospholipids . ARA is distributed rather evenly in gray and white matter and among the different cell types in brain. In contrast, DHA is highly enriched in neuronal membranes including synaptic membranes. Among phospholipids, phosphatidyl-ethanolamine (PtdEtn), plasmenylethanolamine (PlsEtn), and phosphatidylserine (PtdSer) contain high levels of docosahexaenoyl groups (22:6n-3) at the sn-2 position of the glycerol moiety, whereas phosphatidylcholine (PtdCho), phosphatidylinositol (PtdIns), and phosphatidic acid (PtdH) contain high levels of arachidonoyl groups (20:4n-6) (Farooqui et al. 2000b; Tilman and Cascio 2003). Neural membrane phospholipids are hydrolyzed by a group of enzymes called phospholipases. Thus, phospholipase A1 (PLA1) catalyzes the hydrolysis of an ester bond at the sn–1 position forming free fatty acid and 2-acyl lysophospholipid. Phospholipase A2 (PLA2) acts on the ester bond at the sn–2 position liberating free fatty acid and 1-acyl lysophospholipid, which in turn can be acylated by acyl CoA in the presence of an acyltransferase (deacylation/reacylation cycle). Alternatively, a 1-acyl lysophospholipid can be hydrolyzed by a lysophospholipase forming fatty acid and phospho base. Phospholipase C (PLC) hydrolyzes the phosphodiester bond at the sn-3 position of choline glycerophospholipids forming 1,2-diacylglycerols and phosphocholine . Finally, phospholipase D (PLD) cleaves phospholipids into phosphatidic acid and a free base (Farooqui et al. 2000a, b; Farooqui and Horrocks 2007). In neural membranes, phospholipid homeostasis is based on a balance between phospholipid hydrolysis by multiple forms of PLA2, PLC and PLD and resynthesis of phospholipids by the reacylation/deacylation cycle and de novo synthesis pathways (Farooqui et al. 2000a, b). In brain tissue, the catabolism of phospholipids by cPLA2, PLC, and PLD is coupled with neurotransmitter release through dopamine, glutamate, serotonin, P2-purinergic, muscarinic, cytokine, and growth factor receptors. Dopamine, serotonin, and muscarinic receptors are linked through G-proteins where as glutamate receptors do not involve G protein coupling (Farooqui 2009) .
Under physiological conditions ARA is released from PtdCho by isoforms of PLA2. Some ARA is converted to eicosanoids, whereas lyso-phospholipid, the other product of PLA2catalyzed reaction is either converted to platelet activating factor (PAF) or reacylated into brain phospholipids along with ARA (Rapoport 1999). PAF is a potent pro-inflammatory phospholipid mediator with a wide range of biological activities (Farooqui 2011). It acts through G protein coupled receptors (PAF- receptors) and modulates the activation of PLA2, PLC, PLD, cyclooxygenases, and GTPases, along with turnover of phosphatidylinositol, calcium mobilization, and activation of kinases (Farooqui et al. 2008). PAF-mediated neuroinflammation is closely associated with short-and long-term responses of cells to stimulation or neural trauma (Farooqui et al. 2007). PAF promotes adhesive interactions between leukocytes and endothelial cells, leading to transendothelial migration of leukocytes . PAF also modulates the migration of cerebellar granule neurons in cultures (Tokuoka et al. 2003). Intra-carotid infusion of PAF reduces cerebral blood flow with a concomitant increase in the global cerebral metabolic rate for oxygen (Kochanek et al. 1988). Generation of PAF following ischemic injury and disturbance in cerebral blood flow and induction of neuroinflammation support the view that PAF modulates the blood flow in brain tissue (del Zoppo and Mabuchi 2003) .
At low concentration, ARA not only acts as a second messanger itself, but is also a precursor of many lipid mediators (PGs, LTs, TXs, LX) (Table 3.1). ARA not only modulates activities of many enzymes involved in neural cell survival and death, but also modulates ion channels, neurotransmitter release, induction of long-term potentiation, and neural cell differentiation (Farooqui and Horrocks 2007). ARA also acts as a facilitatory retrograde neuromodulator in glutamatergic synapses (Katsuki and Okuda 1995) and modulates acetylcholine release in rat hippocampus (Almeida et al. 1999). Low levels of ARA are also involved in maintaining the structural integrity of neural membranes, determining neural membrane fluidity, and thereby regulating neuronal transmission. However, under pathological conditions, the release of large amounts of ARA not only causes intracellular acidosis and uncouples oxidative phosphorylation, but also results in mitochondrial swelling and production of high levels of ARA-derived lipid mediators through the action of cyclooxygenase-1(COX-1), cyclooxygenase-2 (COX-2) , and lipoxygenases (LOXs) . These mediators include PGs, LTs, TXs, lipoxins, and endocannibinoids (Figs. 3.3 and 3.4) (Wang et al. 2011; Farooqui 2011) .
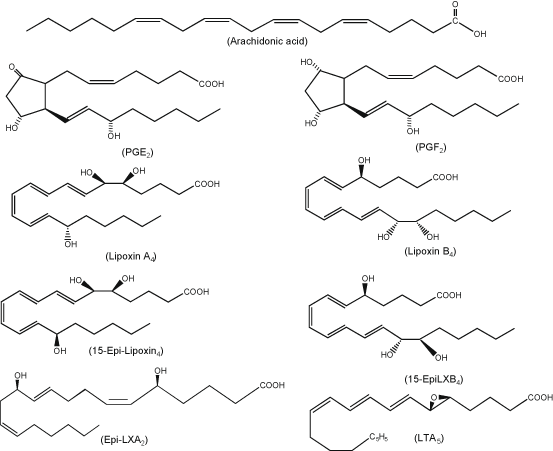
Fig. 3.3
Chemical structures of arachidonic acid-derived lipid mediators.
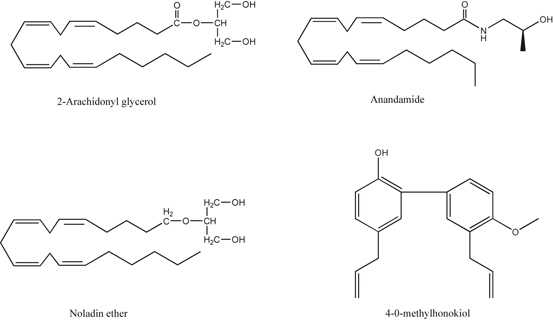
Fig. 3.4
Chemical structures of endocannabinoids and 4-O-methylhonokiol
Table 3.1
Arachidonic acid-derived lipid pro- and antiinflammatory lipid mediators
Substrate | Lipid mediator | Mechanism | Reference |
---|---|---|---|
Arachidonic acid | Prostaglandins | COX-1 & COX-2 | Phillis et al. 2006 |
Arachidonic acid | Leukotrienes | 5-LOX | Phillis et al. 2006 |
Arachidonic acid | Thromboxanes | COX-1 & COX-2 | Phillis et al. 2006 |
Arachidonic acid | Lipoxins | 5-LOX | Chiang et al. 2005 |
Arachidonic acid | 2-Arachidonylglycerol | PLC/DAG-lipase | Farooqui 2011 |
Arachidonic acid | Anandamide | N-Acyltrans-ferase/PLD | Farooqui 2011 |
Table 3.2
Docosahexaenoic acid-derived anti-inflammatory lipid mediators.
Substrate | Lipid mediator | Mechanism | Reference |
---|---|---|---|
Docosahexaenoic acid | Resolvins/docosatrienes | 15-Lipoxygenase | Hong et al. 2003 |
Docosahexaenoic acid | Protectins | 15-Lipoxygenase | Serhan 2005 |
Docosahexaenoic acid | Neuroprotectins | 15-Lipoxygenase | Mukherjee et al. 2004 |
Docosahexaenoic acid | Maresins | 14-Lipoxygenase | Serhan et al. 2009 |
In brain DHA is mainly esterified with PlsEtn and PtdSer. From PlsEtn, it is released by PlsEtn-selective PLA2 . The metabolism of DHA by 15-LOX-like enzyme results in the synthesis of D-series resolvins . 15-LOX also oxidizes DHA into protectin D1 (PD1) through the formation of epoxide intermediate at the 16(17) position. The occurrence of PD1 has also been reported in brain, where it is called as neuroprotectin D1 (10R, 17S-dihydroxy-docosa-4Z,7Z,11E,13E,15Z,19Z-hexaenoic acid, NPD1) (Fig. 3.5). In addition, DHA is also metabolized through a 14-LOX pathway resulting in the generation of 7,14-dihydroxydocosa-4Z,8,10,12,16Z,19Z-hexaenoic acid in macrophages. This metabolite is called as maresin (MaR1). These lipid mediators not only antagonize the effects of PGs, LTs, and TXs, but also modulate leukocyte trafficking and down-regulate the expression of cytokines in glial cells. These metabolites are collectively called as docosanoids. They possess potent anti-inflammatory, neuroprotective and pro-resolving properties (Hong et al. 2003; Marcheselli et al. 2003; Serhan et al. 2005, 2009; Farooqui 2011) .
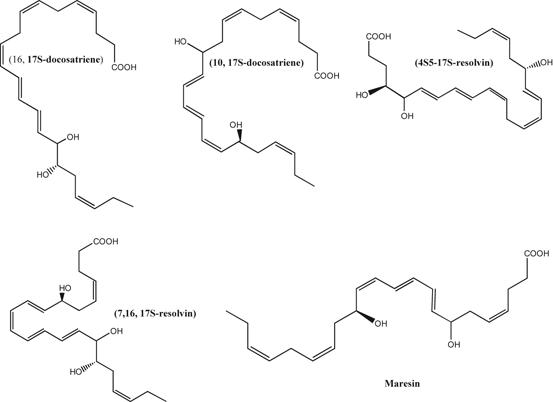
Fig. 3.5
Chemical structures of docosahexaenoic acid-derived lipid mediators
3.4 Arachidonic Acid-Derived Lipid Mediators that Support Neuroinflammation
ARA remains esterified at the sn-2 position of glycerol moiety in neural membrane phospholipids, until mobilized by members of the PLA2 family, hydrolyzing the ester bond in the sn-2 position to yield free ARA and lysophospholipids (Katsuki and Okuda 1995; Phillis et al. 2006; Farooqui and Horrocks 2007). Oxidation of ARA by COXs and LOX transforms this fatty acid (ARA) into PGs, LTs, TXs, and LXs (Fig. 3.3). These metabolites are collectively known as eicosanoids (Phillis et al. 2006). They play important role in the induction of neuroinflammation , oxidative stress , and modulation of various aspects of both innate and adaptive immunity. These lipid mediators are not stored in neural cells, but are synthesized in response to the stimulation of various receptors including dopamine, glutamate, serotonin, muscarinic, cytokine, and growth factor receptors. Because of their amphiphilic nature, eicosanoids can cross cell membranes and leave the cell in which they are synthesized to act on neighboring cells. It is becoming increasingly evident that eicosanoids not only include PGs, LTs, TXs, and LXs, but also hepoxilins and hydroxyeicosatetraenoic (HETE) acids. Among eicosanoids, some PGs and LTs regulate innate and humoral immunity responses, while others induce and modulate inflammation, and are involved in the pathogenesis of various neurological diseases (Phillis et al. 2006). Eicosanoids act through eicosanoids receptors, which are located on plasma and nuclear membranes. Eicosanoid receptors belong to a family of G-protein-coupled receptors that modulate signal transduction pathways and gene transcription. Among eicosanoids, PGs are potent autocrine and paracrine lipid mediators that contribute to physiologic and pathophysiologic responses in the brain. Among various PGs, the most potent are PGD2, PGE2, and PGF2 and PGE2. These PGs mediate their signaling through four distinct G protein-coupled receptors, EP1, EP2, EP3, and EP4, which are encoded by different genes and differ in their responses to various agonists and antagonists and differentially expressed on neuronal and glial cells throughout the central nervous system. The EP1 receptor is coupled to intracellular Ca2+ mobilization and excitotoxic insult-mediated neuronal injury. Whereas EP2 and EP4 receptors are coupled to stimulation of adenylate cyclase via Gs, EP3 receptor is coupled to inhibition of adenylate cyclase via Gi (Minami et al. 2001; Cimino et al. 2008; Furuyashiki and Narumiya 2008; Farooqui 2011). EP2 activation is involved in microglial-mediated paracrine neurotoxicity as well as suppression of microglia internalization of aggregated neurotoxic peptides (Cimino et al. 2008). The activation of EP2 receptor leads to BDNF secretion through stimulation of cyclic AMP dependent signaling involving cAMP-dependent protein kinase (PKA). The catalytic subunit of PKA, stimulates gene transcription through the phosphorylation of cAMP-response element-binding (CREB) protein. This signaling may contribute to neurotoxicity or neuroprotection in microglial cells and astrocytes. EP3 receptor signaling is not only associated with the inhibition of adenylyl cyclase via Gi activation, but is also involved in Ca2+-mobilization through Gβγ from Gi. Along with Gi activation the EP3 receptor can stimulate cAMP formation via G(s) activation. Among EP receptors, EP4 utilizes phosphatidylinositol 3-kinase (PtdIns3K) as well as PKA. In addition, EP4 receptor activates the extracellular signal-regulated kinases (ERKs) 1 and 2 by way of PtdIns3K leading to the induction of early growth response factor-1 (EGR-1), a transcription factor traditionally involved in wound healing and inflammatory processes (Minami et al. 2001; Cimino et al. 2008). Enhanced synthesis of PGE2 in the brain can damage and/or sensitize neurons, resulting in lesions or enhanced pain transmission. However, recent studies have indicated that PGE2 induces anti-inflammatory effects (Brenneis et al. 2011). It induces bradykinin-mediated neuroprotection and blocks LPS- and ATP-induced cytokine synthesis in cultured microglia or in neuron-glia cocultures (Brenneis et al. 2011). The anti-inflammatory and neuroprotective effects of PGE2 are suggested to be mediated via microglial EP2 and EP4 receptors. Mice lacking the EP4 receptor show lower levels of Aβ plaque deposition and less neuronal and synaptic loss than control mice (Hoshino et al. 2012). Oral administration of a specific EP4 receptor antagonist, AE3-208 to APP23 mice not only improves cognitive performance and decreases brain levels of Aβ, but also suppresses endocytosis and activation of γ-secretase (Hoshino et al. 2012) indicating that inhibition of the EP4 receptor improves the cognitive function of APP23 mice by suppressing Aβ production and reducing neuronal and synaptic loss. Under normal conditions PGs, LTs, and TXs and their receptors regulate neural activity by releasing neurotransmitters and modulating circulatory function (Phillis et al. 2006). However, under pathological conditions the generation and accumulation of PGs, LTs, TXs, and LXs regulate cerebrovascular blood flow through the modulation of vasoconstriction and vasodialation. The active production PGs, LTs, TXs, and LXs by circulating cells such as platelets and leukocytes may contribute to the onset of alterations in the microcirculation and ultimately to CNS dysfunction (Phillis et al. 2006). High levels of PGs have degenerative affects on differentiated murine neuroblastoma cells in cultures. In vivo, PGs are involved in the regulation of cytokines and maintenance of the inflammatory cascade (Phillis et al. 2006; Farooqui et al. 2011).
3.4.1 Metabolism and Role of Prostaglandins in Neuroinflammation
Four major PGs are known to occur in the brain . They include PGE2, prostacyclin (PGI2), prostaglandin D2 (PGD2) and prostaglandin F2α (PGF2α) (Ogorochi et al. 1984). Formation of PGs depends on the activity of cyclooxygenase-1 and cyclooxygenase-2 (COX-1 and COX-2) or prostaglandin G/H synthases. They are bifunctional enzymes, which contain both cyclooxygenase and peroxidase activity. COX-1 is expressed constitutively in most neural and non-neural cells. It is the dominant source of PGs for housekeeping functions. In contrast, COX-2 is induced by inflammatory stimuli, neurotransmitters, hormones and growth factors and is a very important source of PGs formation in the neuroinflammation and in the proliferative diseases, such as cancer (Dubois et al. 1998; Farooqui 2011). PGH2 is synthesized by both COX-1 and COX-2 and it is the common substrate for a series of specific isomerase and synthase enzymes that produce PGE2, PGI2, PGD2, PGF2α and TXA2. COX-1 activity is preferentially coupled with thromboxane synthase (TXS), prostaglandin F synthase, and the cytosolic (c) prostaglandin E synthase (PGES) isozymes. In contrast, COX-2 is coupled with prostaglandin I synthase and the microsomal (m) PGES isozymes (Smyth et al. 2009). The generation of PGs and LTs not only contribute to the initiation, maintenance, and development of neuroinflammation, but also promotes edema formation and oxidative toxicity. The biosynthesis and release of the potent chemotactic agent (LTB4) promotes the recruitment of neutrophils (PMNs) to the inflamed tissue, while the formation of PGE2 and PGD2 further accelerates the inflammatory process, ultimately resulting in so called acute inflammation .
Among PGs, PGD2 and PGE2 are unstable, and readily undergo in vivo and in vitro non-enzymic dehydration to generate the biologically active cyclopentenone J2 prostaglandins, which include PGJ2, Δ12-PGJ2 and 15-deoxy-Δ12, 14-PGJ2 (15d-PGJ2) and PGA2, respectively (Fig. 3.6) (Shibata et al. 2002). Unlike other PGs, both PGA2 and PGJ2 contain an unsaturated carbonyl moiety in their cyclopentenone ring structure, which is highly reactive and can readily form Michael adducts with nucleophilic substrates, such as thiol groups. The formation of cyclopentenone eicosanoids (PGJ2, PGA2) in the brain may represent a novel pathogenic mechanism that contributes to many neuro-degenerative conditions (Musick et al. 2005; Farooqui 2011). 15-deoxy-δ12,14-PGJ2 is a high affinity ligand for the nuclear receptor PPARγ. It modulates gene transcription by binding to this receptor. Other activities of the cyclopentenone prostaglandins are facilitated by the reactive α, β-unsaturated carbonyl group located in the cyclopentenone ring .
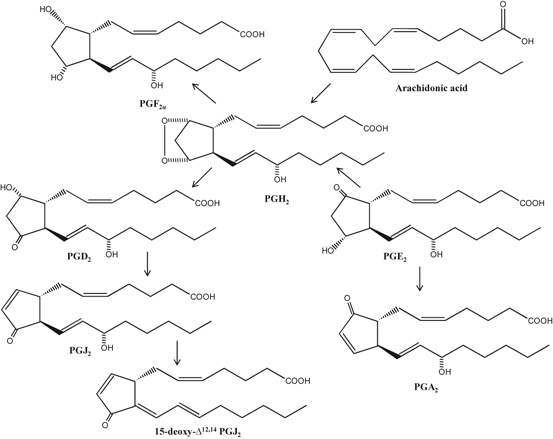
Fig. 3.6
Chemical structures of arachidonic acid-derived prostaglandins
NF-κB is a redox sensitive transcription factor that most commonly exists as a p50/p65 heterodimer. This heterodimer is localized in the cytoplasm when associated with inhibitor of kappa B (IκB) proteins. Upon stimulation (e.g. by TNFα) IκB proteins get phosphorylated by upstream IκB kinases (IKKs) followed by degradation, releasing the active dimer to translocate into the nucleus to transcribe its target genes (Hayden and Ghosh 2004). NF-κB and its activating kinase (I-κB kinase inhibition and blockade of NF-κB nuclear binding) are major targets for the anti-inflammatory activity of 15d-PGJ2, which blocks NF-κB-induced transcriptional activation by PPARγ-dependent and independent molecular mechanisms. Thus, 15d-PGJ2 inhibits the expression of iNOS and TNFα in several cell types that are dependent on PPARγ (Ricote et al. 1998). However, some anti-inflammatory responses of 15d-PGJ2 that are PPARγ independent (Vaidya et al. 1999) . Based on detailed studies, it is proposed that 15d-PGJ2 is a very stable metabolite that can diffuse between cells within a tissue to mediate the resolution of inflammation (Gilroy et al. 1999). Important key steps during resolution of inflammation are an increase in local vascular permeability leading to edema (Shimizu 2009; Farooqui 2011) and the transport of n-3 fatty acids from blood to the site of inflammation (Kasuga et al. 2008). At the site of inflammation, n-3 fatty acids are converted to novel potent mediators by exudate leukocytes that promote resolution of inflammation (Kasuga et al. 2008; Farooqui 2011). There are studies that report protective effects mediated by 15d-PGJ2 via inhibition of infiltration of immune cells in various models of inflammation e.g. endotoxic shock (Kaplan et al. 2005), and ischemia/reperfusion injury (Cuzzocrea et al. 2003) and experimental autoimmune encephalomyelitis (EAE) (Diab et al. 2002) . Thus, based on these studies, it has been hypothesized that 15d-PGJ2 inhibits the adhesion of mononuclear cells to the endothelial cells and thereby attenuates their transmigration. Many of 15d-PGJ2 metabolites not only possess anti-neoplastic, but also induce anti-viral activities (Straus and Glass 2001). Unlike other PGs, which produce their effects by interacting with membrane-bound EP receptors, cyclopentenone PGs are taken up by cells via an active transport process and accumulate intracellularly (Narumiya and Fukushima 1986) with nearly 50 % of the compound transported to the nucleus and act as potent repressors of cell growth and inducers of cell differentiation (Narmiya et al. 1987; Negishi et al. 1995) . Cyclopentenone PGs are rapidly inactivated by glutathione (GSH) in cells via glutathione transferase (GST)-mediated conjugation (Bogaards et al. 1997) and then discarded from the cell by the action of ATP-dependent efflux pumps (Paumi et al. 2003). Variability between cell types in GSH and GST levels and efflux pump activity may explain the differential susceptibility of various cell lines to the effects of cyclopentenone PGs (Homen de Bittencourt and Curi 2001). Accordingly, depletion of intracellular GSH levels potentiates the effects of cyclopentenone PGs, while augmentation of cellular GSH content protects cells from these compounds (Levonen et al. 2001) .
15d-PGJ2 exhibits protective effects against oxidative stress . This effect of 15d-PGJ2 may be due to the activation of Keap1-Nrf2-ARE pathway (Fig. 3.7). Nuclear factor (erythroid-derived 2)-like 2 (Nrf2) is a member of the leucine zipper transcription factor family. It is localized in the cytoplasm in a bound form with Kelch-like ECH-associated protein 1 (Keap1). Phosphorylation of Kelch-like ECH-associated protein 1 (Keap1) not only results in the dissociation of Nrf2 from Keap1, but also in translocation of Nrf2 into the nucleus. Nrf2 binds to an antioxidant response element (ARE) and modulates the expression of many genes including genes associated with antioxidant-detoxifying proteins, genes involved in cellular rescue pathways against oxidative injury, inflammation/immunity, apoptosis, and carcinogenesis. ARE-driven genes include direct antioxidants (glutathione peroxidases), thiol metabolism-associated detoxifying enzymes (glutathione S-transferase), stress-response genes (hemeoxygenase), quinine reductase, and others (proteasome subunit beta type-5). Collective evidence suggests that 15d-PGJ2 not only protects cells from endogenous and exogenous stresses, but also induce anti-inflammatory effects (Gayarre et al. 2007; Kansanen et al. 2009) .
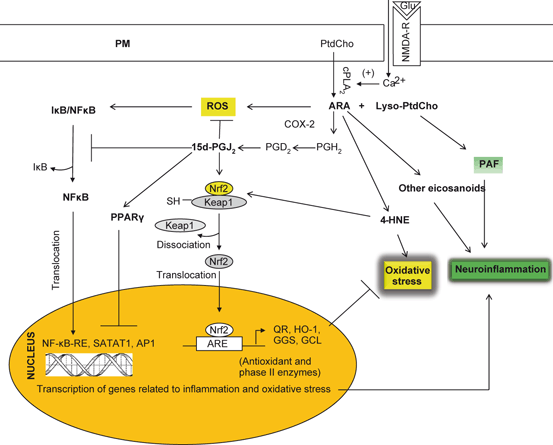
Fig. 3.7
Hypothetical signal transduction diagram showing antioxidant mechanism induced by 15d-PGJ2. Plasma membrane (PM), N-Methyl-D-aspartate receptor (NMDA-R), Glutamate (Glu), Phosphatidylcholine (PtdCho), cytosolic phospholipase A2 (cPLA 2 ), lysophosphatidylcholine (lyso-PtdCho), arachidonic acid (ARA), platelet activating factor (PAF), 4-hydroxynonenal (4-HNE), cyclooxygenase-2 (COX-2), prostaglandin D2 (PGD 2 ), rective oxygen species (ROS), Kelch-like ECH-associated protein 1 (Keap1), nuclear factor-erythroid-2-related factor 2 (Nrf2), nuclear factor-κB (NF-κB), nuclear factor-κB-response element (NF-κB-RE), inhibitory subunit of NF-κB (I-κB), heme oxygenase (HO-1), γ-glutamate cystein ligase (γ-GCL)
3.4.2 Metabolism and Role of Leukotrienes in Neuroinflammation
LOXs are non-heme iron containing dioxygenases involved in the oxygenation of arachidonic acid and other polyunsaturated fatty acids . Depending on the position of insertion of oxygen, LOXs are classified into 5-, 8-, 9-, 12- and 15-LOX. Among various isoforms, 5-LOX is the most predominant enzyme, which is associated with the formation of 5-hydroperoxy-eicosatetraenoic acid (5-HpETE), the precursor of non-peptido (LTB4) and peptido (LTC4, LTD4, and LTE4) leukotrienes. The first step in the biosynthesis of LTs is the conversion of ARA to LTA4 by 5-LOX, which catalyzes a two-step reaction with 5-hydroperoxy-eicosatetraenoic acid as an intermediate, which is instantaneously, further converted to LTA4 (Peters-Golden and Henderson 2007; Radmark and Samuelsson 2009). 5-LOX migrates from the cytosol to the nuclear membrane to associate with the 5-LOX-activating protein, which presents bound ARA to 5-LOX (Evans et al. 2008). LTA4 serves as substrate for the generation of all bioactive LTs. Brain contains only three forms of LOX (5-LOX, 12-LOX, and 15-LOX) (Phillis et al. 2006). Of these, 12-LOX is the most abundant isoform found in the brain (Hambrecht et al. 1987), with significant mRNA expression in rat cortical neurons, astrocytes, and oligodendrocytes (Bendani et al. 1995). 5-LOX activity requires a small 18 kDa protein known as FLAP (5-LOX activating protein) for leukotriene synthesis (Byrum et al. 1997). Evidence suggests that FLAP binds ARA for presentation to 5-LOX (Mancini et al. 1993). The LOX-mediated metabolites of ARA serve as second messengers and modulate neuroinflammation, apoptosis, and synaptic activity in the brain. 5-LOX metabolizes ARA into LTA4. This unstable intermediate is then metabolized by LTA4 hydrolase or into LTC4 by LTC4 synthase (Borgeat et al. 1976; Radmark et al. 1980; Phillis et al. 2006). Cysteinyl leukotrienes (cysLTs) (LTC4, LTD4, LTE4) are an important class of proinflammatory lipid molecules (Fig. 3.8), which are potent biological mediators in the pathophysiology of inflammatory diseases. LTs are involved in many innate immunological processes such as leukocyte adhesion, chemotaxis and activation (Samuelsson 1983; Funk 2001). They regulate the immune response to different stimuli, and are known to play an important role in nonneural (asthma, vascular disease, and cancer) and neurological conditions (stroke, Alzheimer disease, and Parkinson disease) (Phillis et al. 2006; Peters-Golden and Henderson 2007). In addition to potent proinflammatory activities, LTs display strong vasoconstrictive, chemotactic and proliferative properties. Among LTs, LTB4 is known as a potent chemoattractant. It not only induces the formation of reactive oxygen species , but also facilitates the release of lysosome enzymes from leukocytes. Known as the slow-reacting substances of anaphylaxis, cysLTs (LTC4, LTD4, LTE4) induce inflammation, and may also, when in excess, induce anaphylactic shock (Peters-Golden and Henderson 2007). CysLTs mediate their effects through interactions with two types of G-protein coupled receptors (GPCR): CysLT type 1 receptor (CysLT1) and type 2 receptor (CysLT2) . These receptors differ from each other in binding affinities for different cysLTs. CysLT1 is recognized as a high-affinity receptor for LTD4, whereas CysLT2 binds LTC4 and LTD4 with similar affinity (Foster et al. 2013). LTE4 is the least active cysLTs and has low affinity for binding to the classical receptors and lowest functional agonistic potency in comparison to LTC4 and LTD4. Neurochemical effects of LTs are transduced through highly conserved G protein coupled receptors (GPCRs), which are named as BLT1 and BLT2 receptors (Yokomizo et al. 2000). LTB4 shows high affinity for BLT1 (K d , ~ 0.5 nM), whereas the affinity to BLT2 receptor is considerably lower (K d , ~ 23 nM) (Kim and Luster 2007). Activation of BLT1 and BLT2 receptors increases intracellular Ca2+ levels, which activates PLC and decreases cAMP levels through the inhibition of adenylyl cyclase activity (Peter-Golden and Henderson 2007). LTB4–BLT interactions play important roles in host defense mechanism and inflammatory diseases. Mice lacking in leukotriene production are insensitive to some inflammatory stimuli, and mice overexpressing BLT1 exhibit enhanced responses to infections and lung ischemic-reperfusion injury (Chiang et al. 1999).
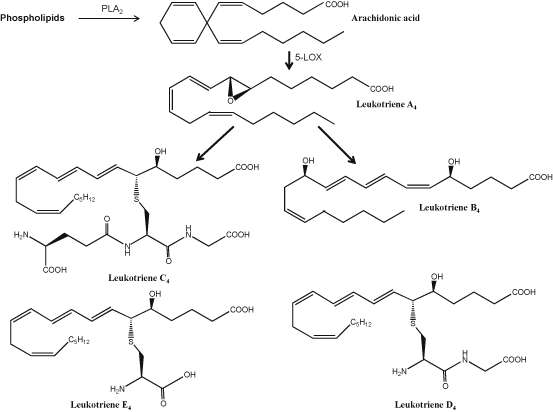
Fig. 3.8
Chemical structures of arachidonic acid-derived leukotrienes
3.4.3 Metabolism and Role of Thromboxanes in Neuroinflammation
As stated above, action of COX-1 on ARA converts it to PGH2, which is transformed into thromboxane A2 (TX2) by thromboxane synthase (Needleman et al. 1976) . Thromboxanes produce their effects through GPCRs. These receptors are called as thromboxane receptors (TPRs) (Mir and Le Breton 2008). Two splice variants of TPRs have been reported to occur. One cloned from human placenta and megakaryocytic lines (TPRα) and the other from human endothelial cells (TPRβ). TPRα and TPRβ differ in the length of their carboxyl-terminal extensions (15 versus 79 residues), which contain multiple potential sites for receptor phosphorylation (Habib et al. 1997). There are clear differences between the mechanisms of downstream signaling of two varients of TPRs. Both TPRα and TPRβ are coupled to downstream signaling pathways via interaction with predominantly Gq11 type of G protein. They are not only associated with the activation of protein kinase C, RhoA (Nie et al. 2008) and AMP-activated protein kinase (Zhang et al. 2008), but can also stimulate release of intracellular calcium stores. Regulation of TPR signaling is very complex. It not only involves multiple kinase-mediated pathways that are associated with receptor desensitization, but is also coupled with oligomerization of TPR splice variant (Laroche et al. 2005; Wilson et al. 2007). TXs and TPRs are expressed in the developing rat brain during myelination (Mir and Le Breton 2008). Furthermore, culture of oligodendrocytes (OLG) progenitor cells (OPCs) show that the expression levels of these proteins as well as TXA2 synthesis increase during OLG maturation . In cardiovascular and cerebrovascular systems the activation of TPRs is associated with potent vasoconstriction, which contributes to increased vascular tone, severe unstable angina, inflammation, and blood pressure (Liu et al. 2010b). Based on detailed investigations, it is suggested that activation of TPRs impair cyclic AMP-dependent vasorelaxations partly via PDE- and RhoA/Rho kinase-dependent mechanisms and inhibitors of PDEs and Rho kinase can be useful in the treatment of cardiovascular and cerebrovascular complications (Liu et al. 2010b) .
3.4.4 Metabolism and Role of Lipoxins in Suppression of Neuroinflammation
Lipoxins (LXs) are a group of endogenous trihydroxytetraene eicosanoids, which are synthesized by the action of LOXs on hydroperoxyeicosatetraenoic acid (HPETE) and hydroxyeicosatetraenoic acid (HETE). LXs include 5S,6R,15S-trihydroxy-(7E,9E,11Z,13E)-tetraenoic acid (LXA4), 5S,14R,15S-trihydroxy-6E,8Z,10E, 12E-eicosatetraenoic acid (LXB4), 15 epi-LXA4, and 15 epiLXB4 (Fig. 3.3). As the first recognized class of anti-inflammatory lipid-based autacoids, LXs mediate a number of processes, including the regression of pro-inflammatory cytokine production, inhibition of cell proliferation, promotion of the recruitment of monocytes and stimulation of non-phlogistic phagocytosis of apoptotic leukocytes by monocyte-derived macrophages, suggesting that lipoxins may act as endogenous ‘braking signals’ in host defense, inflammation and hypersensitivity reactions (Yacoubian and Serhan 2007; Serhan et al. 2011). The synthesis of LXs has been extensively studied in non-neural tissues (Romano 2010). The first pathway of LXs biosynthesis involves the interaction of platelets with PMN within the vascular lumen. In this pathway, biosynthesis of LXs starts with the release of the epoxide intermediate LTA4 formed by 5-LOX in activated leukocytes, which is then converted by the platelet 12-LOX to LXA4 and LXB4 (Serhan and Sheppard 1990; Serhan and Romano 1995). The second pathway of transcellular LXA4 biosynthesis involves the sequential interaction of a 15-LOX with a 5-LOX. In this pathway, the synthesis of LXA4 takes place mainly in tissues in which endothelial and epithelial cells expressing 15-LOX can interact with 5-LOX-containing leukocytes (Chiang et al. 2005) (Fig. 3.9). The third pathway of LX biosynthesis is initiated by aspirin, which acetylates COX-2 and switches its catalytic activity from COX to 15-LOX. Following this change, PG biosynthesis is inhibited and COX-2 transforms ARA to 15(R)-HETE. 15(R)-HETE is subsequently transformed by activated leukocytes possessing 5-LOX to a new series of carbon-15 epimers of LXA4 that carry their 15 alcohol in the R configuration (15-epi-LXA4) (Fig. 3.9) (Claria and Serhan 1995; Serhan 2005; Chiang et al. 2005). Under Normal conditions, LXs are generated by transcellular metabolism between neutrophils, platelets, and resident tissue cells, such as epithelial cells (Serhan 2007), through the sequential action of 5-LOX and either 12-LOX or 15-LOX (Serhan 2005). Biosynthesis of LXs in the tissue not only depends on cells and enzymes present therein, but also on other factors such as cytokines (Serhan 2005). For example, interleukins (IL-4 and IL-13), putative negative regulators of inflammatory and immune responses, promote transcellular LX generation through enhanced expression of 15-LOX in blood monocytes and epithelial cells (Munger et al. 1999). IL-3 upregulates 5-LOX transcript (Murakami et al. 1995) while IL-1β, IL-6 and TNF-α induce COX-2, thus potentially contributing to the formation of aspirin triggered lipoxins in vivo (Parente and Perretti 2003). LXs mediate their actions through receptors. These receptors are called as ALX receptor. It has been recently cloned (Romano et al. 2007).
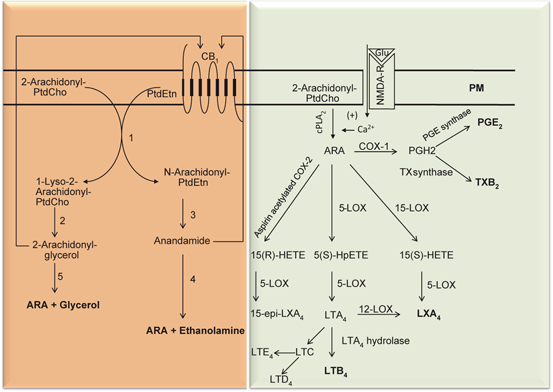
Fig. 3.9
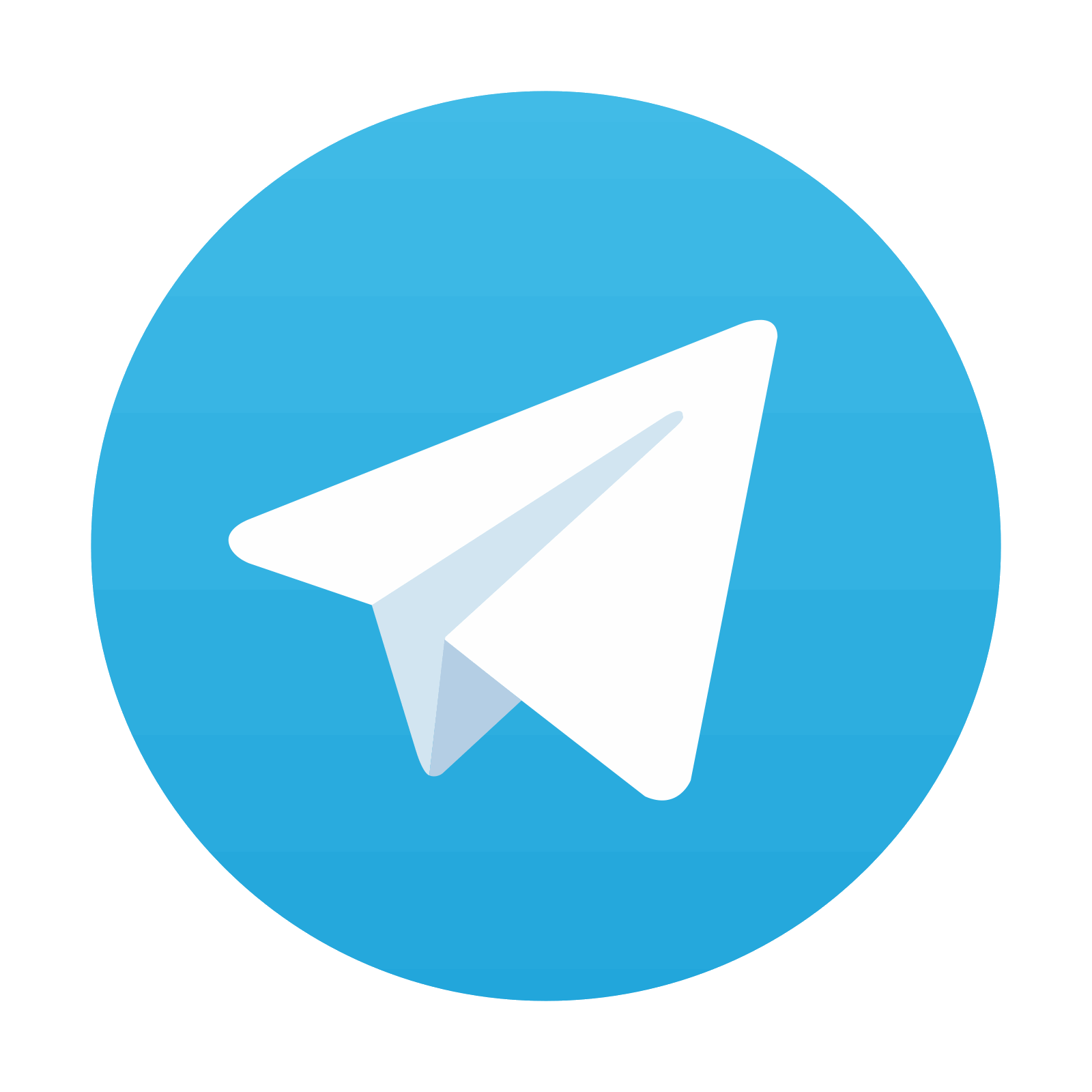
Synthesis of prostaglandins, leukotrienes, and lipoxins from arachidonic acid. Plasma membrane (PM), N-Methyl-D-aspartate receptor (NMDA-R), cannabinoid receptor 1 (CB1), Glutamate (Glu), Phosphatidylcholine (PtdCho), cytosolic phospholipase A2 (cPLA 2 ), lysophosphatidylcholine (lyso-PtdCho), arachidonic acid (ARA), 5-lipoxygenase (5-LOX), 12-lipoxygenase (12-LOX), cyclooxygenase (COX), phosphatidylethanolamine (PtdEtn), lipoxin A4( LXA 4 ), 15-epi-lipoxin A4 (15-epiLXA 4 ), and various leukotrienes (LTA 4 and LTB 4 )
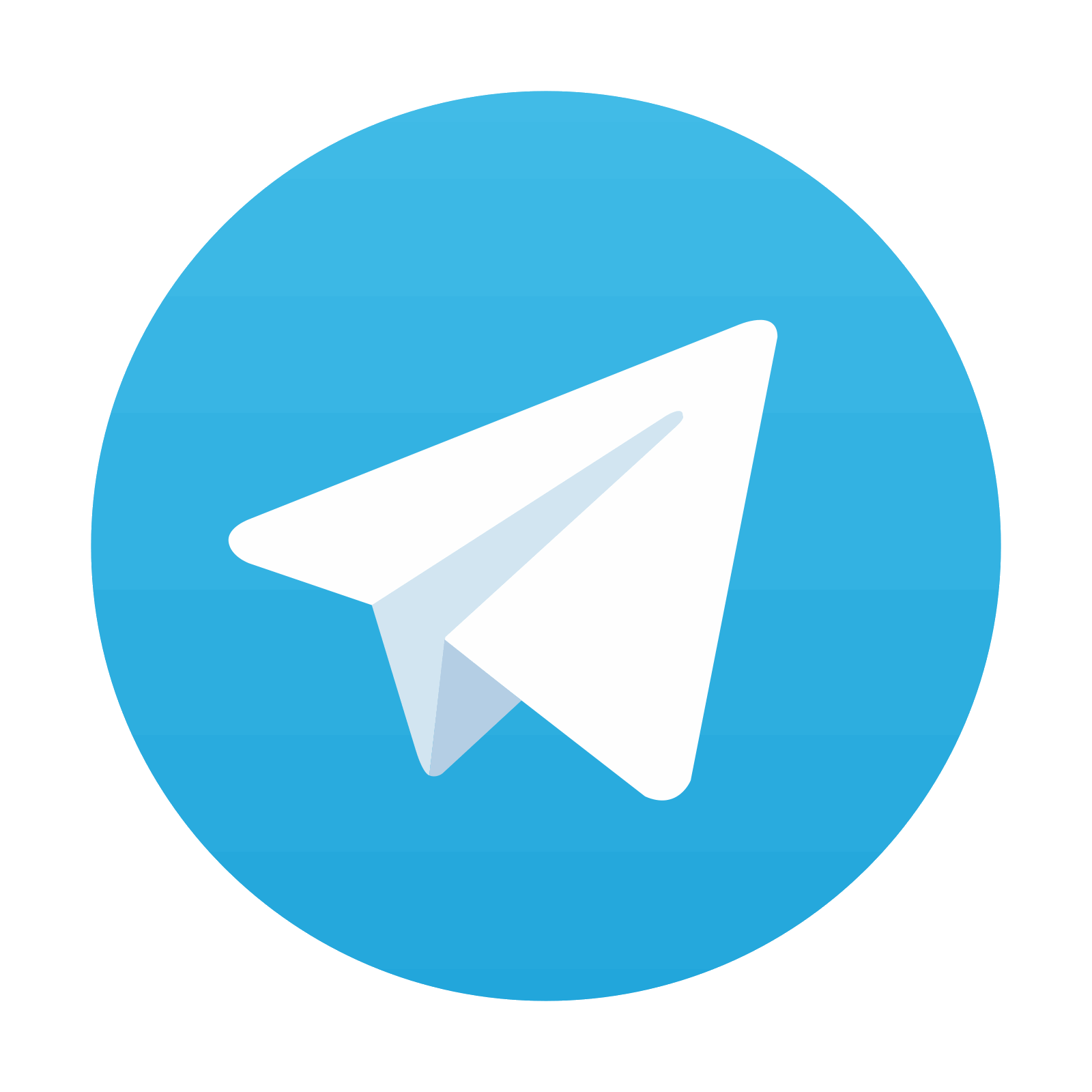
Stay updated, free articles. Join our Telegram channel
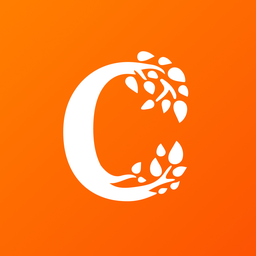
Full access? Get Clinical Tree
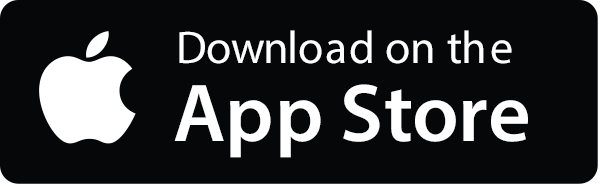
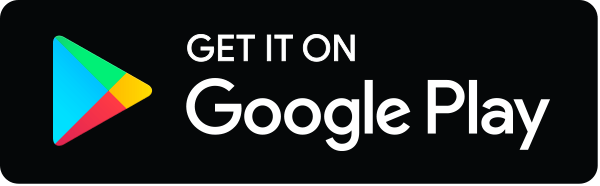
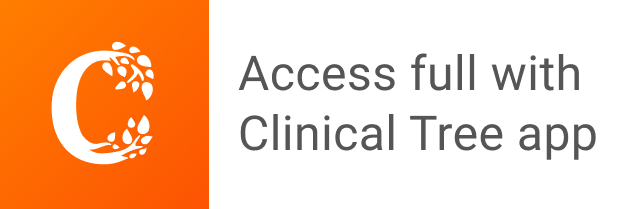