Fig. 11.1
Consumption of Western diet suppresses adaptive responses
Physical inactivity and lack of sleep are strongly associated with coronary heart disease (Powell et al. 1987), type II diabetes (Helmrich et al. 1991; Manson et al. 1992), metabolic syndrome, and neurological disorders (Farooqui et al. 2012; Farooqui 2013). Thus, improvements in dietary habits, physical activity, and sleep can reduce the risk of developing chronic visceral and neurological disorders (Farooqui 2013). Despite of substantial evidence linking diet, exercise , and sleep with favorite health outcomes, the great majority of Americans do not meet dietary, physical activity, and sleeping time guidelines (Serdula et al. 2004). More than half of US adults do not get enough physical activity and sleep to provide optimal health benefits, including approximately one-fourth who are sedentary (Leavitt 2008). Similarly, only one-fourth of US adults consume 5 or more fruits and vegetables per day (Leavitt 2008). These results strongly suggest that good nutrition, exercise, and regular sleep are necessary for optimal health outcome (Tochikubo et al. 1996).
11.2 Effect of Diet on Oxidative Stress
As stated above, Western diet is enriched in saturated fat, cholesterol, arachidonic acid (ARA), and refined sugar. In addition, Western diet is low in fiber and is loaded with high salt. Long-term consumption of Western diet causes an increase in the generation of ARA-derived lipid mediators such as prostaglandins (PGs), leukotrienes (LTs), thromboxanes (TXs), and lysophospholipid-derived platelet activating factor. These lipid mediators contribute to oxidative stress and proinflammatory responses in the brain (Farooqui 2010, 2011, 2013). The presence of high levels of saturated fats, cholesterol, and ARA-derived lipid metabolites produces cognitive decline through the reduction in molecular substrates that support cognitive function and increase the risk of neurological dysfunction through the generation of PGs, LTs, and TXs in both humans and animals (Farooqui 2009). In addition, consumption of high energy density food in Western diet promotes weight gain and obesity, and insulin resistance in brain and visceral tissues. These processes result in short lifespan and accelerate the onset of age-related disorders, including diabetes, cancer and neurological disorders (stroke, AD, and depression) (Fig. 11.2) (Farooqui 2013). Long-term consumption of Western diet also induces oxidative stress, mitochondrial abnormalities, and facilitates the formation of protein aggregates (Aβ aggregation in AD and Lewy bodies and lipofuscin accumulation in PD), but also reduces the levels of trophic factors, and promote the induction of neuroinflammation. These changes may cause functional impairments in the brain (Sakano et al. 2009; Farooqui 2010, 2013) .
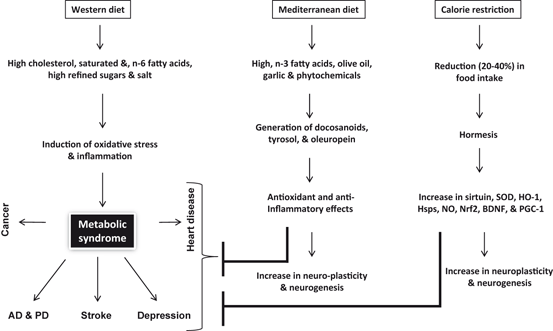
Fig. 11.2
Effects of Western and Mediterranean diet and calorie restriction on the pathogenesis of neurological disorders. Alzheimer disease (AD), Parkinson disease (PD), superoxide dismutase (SOD), heme oxygenase (HO-1), heat shock proteins (Hsp), nitric oxide (NO), nuclear factor (erythroid-derived 2)-like 2 (Nrf2), brain-derived neurotrophic factor (BDNF), and peroxisome proliferator-activated receptor gamma coactivator 1-alpha (PGC-1α)
In contrast, the Mediterranean diet is not only high in fresh fruits, green vegetables, olive oil, legumes, and fish, but also has high fiber contents and enriched in oleic acid (OA), antioxidants (tyrosol and hydroxytyrosol) and docosahexaenoic acid (DHA). 15-Lipoxygenase (15-LOX)-mediated oxidation of DHA results in the synthesis of docosanoids such as neuroprotectins, resolvins, and maresins, which produce antioxidative, anti-inflammatory and pro-resolutionary effects (Farooqui 2009; Farooqui 2011). In humans, DHA enriched diet also supports cognitive processes due to increase in neuroplasticity . Similarly, in rodents consumption of DHA-enriched diet modulates genes that support and maintain of synaptic function and neuroplasticity by facilitating neurogenesis , synaptogenesis, cortical re-organization (Farooqui 2009). The ability of DHA to modulate cognitive function may also be associated with its capacity to modulate energy metabolism. In addition, DHA also modulates mitochondrial genes exepression and mitochondrial biogenesis (Farooqui 2009). Due to the presence of olive oil, garlic, red wine, and fresh fruits (flavonoids and vitamins), consumption of Mediterranean diet produces antioxidant effects, which promote beneficial effects on neurovascular and cardiovascular systems (Farooqui 2012) (Fig. 11.2). Olive oil in Mediterranean diet increases brain glutathione levels suggesting reduced oxidative stress as a possible mechanism. In addition, effect of olive oil is accompanied by increase in glutathione reductase, superoxide dismutase activities, and decrease in tissue levels of 4-hydroxynoneal and 3-nitrotyrosine. These observations suggest that olive oil produces beneficial effects not only due to its antioxidant activities, but also due to its high content of nitrate-rich leafy green vegetables, which are known to lower the blood pressure of healthy volunteers (Lundberg et al. 2006; Larsen et al. 2006). A possible link between telomere length or maintenance and consumption of Mediterranean diet has been also recently reported (Boccardi et al. 2013). These observations support the view that adherence to Mediterranean-diet correlates to higher longevity and healthy aging not only due to the inhibition of oxidative stress and inflammation, but also reduction in cellular senescence, apoptosis and maintenance of telomere’s length (Haveman-Nies et al. 2003; Marin et al. 2011). Collective evidence suggests that the Mediterranean diet provides protection against cognitive decline in older individuals since it combines several foods and nutrients potentially protective against cognitive dysfunction, dementia, and neurodegeneration. These nutrients include n-3 fatty acid in fish, monounsaturated fatty acids, vitamins B12, and folic acid, along with other antioxidants in olive oil, nitrate in green vegetables and moderate amounts of resveratrol in red wine (Scarmeas et al 2006). In vitro and in vivo studies have shown that these dietary factors can act through several mechanisms, such as cell signaling and modulation of gene expression, reduction in oxidative stress, inhibition of inflammatory mediator production, and retardation of lipid accumulation (Farooqui 2013). Despite these effects, information from observational and human intervention studies remains controversial and has failed to demonstrate that addition of a single dietary component reduces pathologies associated with oxidative stress and inflammation (Farooqui 2013). It is, therefore, likely that beneficial effects mediated with consumption of above mentioned diet may involve the cumulative effects of multiple nutrients .
Calorie restriction also produces beneficial effects on brain health. Beneficial effects of calorie restriction on the brain are mediated through the activation of adaptive cellular stress responses, in a process called hormesis (Cornelius et al. 2013; Mattson 2008). At the molecular level, calorie restriction reduces the generation of reactive oxygen species (ROS) and nitrogen species (RNS) in mitochondria. The molecular mechanism involved in this process is not fully understood. However, it is proposed that under calorie restriction, sirtuin (SIRT3) deacetylates and subsequently activates SOD2, leading to reduction in oxidative stress (Chen et al. 2011) (Fig. 11.3). In addition, calorie restriction is accompanied by the upregulation of adaptive stress response proteins in neurons (Martin et al. 2006). Reducing energy intake by controlled caloric restriction not only increases lifespan (by 20–40 %) and protects neural cells against neurological disorders, but also enhances cellular stress resistances. Calorie restriction also decreases aging-related learning and memory impairments in animals and humans (Witte et al. 2009), probably through higher expression of an NMDA-receptor subunit in the hippocampus. In addition, calorie restriction not only attenuates age-related brain atrophy in monkeys (Colman et al. 2009), but also stabilizes the expression of synaptic protein expression to avoid aging-related changes (Mladenovic Djordjevic et al. 2010) .
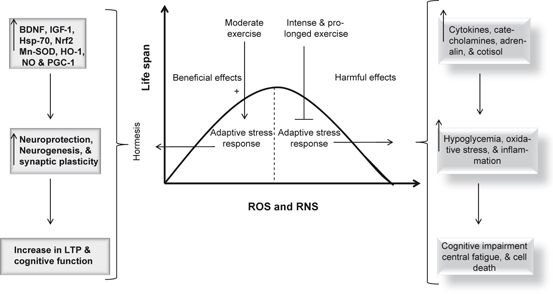
Fig. 11.3
Effect of oxidative and nitrosative stress on life span. Superoxide dismutase (SOD), heme oxygenase (HO-1), heat shock protein-70 (Hsp-70), nitric oxide (NO), nuclear factor (erythroid-derived 2)-like 2 (Nrf2), brain-derived neurotrophic factor (BDNF), and long term potentiation (LTP)
11.3 Effect of Diet on Neuroinflammation
Long-term consumption of Western diet also produces neuroinflammation, a process, which is supported by the generation of eicosanoids (Farooqui 2010) from arachidonic acid (ARA), a major component of Western diet (Farooqui 2013). In addition, the non-enzymic oxidation of ARA also produces ROS and 4-hydroxynonenal (4-HNE). These metabolites facilitate the migration of transcription factor NF-κB from cytoplasm to the nucleus (Karin and Ben-Neriah, 2000) where it facilitates the expression of a number of proteins including many proinflammatory enzymes (sPLA2, COX-2, NADPH oxidase and inducible nitric oxide synthase) and proinflammatory cytokines (TNF-α, IL-1β, and IL-6) (Farooqui 2009, 2010). Elevated levels of these enzymes, cytokines along with the generation of eicosanoids and platelet activating factor promote and maintain inflammation in the brain. Oxidative stress and inflammation appear to be closely interlinked with processes in neurological disorders, although it is difficult to establish the temporal sequence of their relationship. For example, several proinflammatory transcription factors , including nuclear factor-κB (NF-κB) and activator protein-1 (AP-1) , are redox sensitive; therefore, ROS trigger the release of inflammatory cytokines, which in turn enhance ROS production, thus establishing a vicious circle (Farooqui 2013). In addition, chronic and sustained nature of neuroinflammation produces alterations in blood brain barrier (BBB) permeability leading to increase in infiltration of peripheral macrophages into the brain parenchyma to further perpetuate the neuroinflammation.
Chronic inflammation associated with neurodegenerative and neuropsychiatric diseases involves a network of neuronal and glial cell that integrate many complex signaling pathways (Farooqui 2013). Mediators of these pathways not only include major stress hormones (noradrenaline and adrenaline and cortisol); pro-inflammatory cytokines (TNF-α), interleukin (IL)-6 and IL-1β); free fatty acids, and, oxidized phospholipids (Farooqui and Horrocks 2007). Recent reports on the effect of Western diet have indicated that this diet produces inflammation both in the peripheral tissues and hypothalamus, a brain region, which is critical for the maintaining energy homeostasis (Thaler et al. 2012) . Unlike peripheral inflammation, which develops as a consequence of overeating Western diet and induction of insulin resistance after weeks to months, the onset of hypothalamic inflammation occurs both in rats and mice within 1–3 days after the start of high fat and high sugar diet and prior to substantial weight gain. Hypothalamic inflammation is accompanied by reactive gliosis involving both microglial and astroglial cell populations along with increase in markers of neuron injury (TNF-α, IL1-β, and IL-6) within a week. These studies on rodents are supported by MRI studies in humans, which indicate that consumption of Western diet increases gliosis in the mediobasal hypothalamus of obese humans. Collective evidence suggests that in both humans and rodents the consumption of Western diet is associated with neuronal injury in the hypothalamus, an area of the brain involved in body weight control (Thaler et al. 2012). Limited information is available on the molecular mechanisms associated with neuronal injury after long term consumption of Western diet. However, based on several studies, it is proposed that the presence of high fat and high sugar in the Western diet induces inflammation in both peripheral tissues and hypothalamus through the activation of TLR4 receptors, IKKβ/NF-κB signaling, and induction of SOCS3 along with induction of endoplasmic reticulum stress, (Thaler and Schwartz 2010; Thaler et al. 2012; Fessler et al. 2009; Zhang et al. 2008). More studies are needed on the molecular mechanism of harmful effects of Western diet on human brain and visceral tissues .
As stated above, the consumption of Mediterranean diet results in the synthesis of docosanoids, which produce anti-inflammatory effects throughout the body including brain. Mediterranean diet lowers neuroinflammation not only due to the production of docosanoids, but also due to the presence of anti-inflammatory phytochemicals found in fresh fruits and sulfur compound of garlic (Perez-Jimenez et al. 2007; Lopez-Miranda et al. 2007; Farooqui 2012). Similarly calorie restriction produces beneficial effects through hormesis , a process, which not only inhibits oxidative stress and neuroinflammation through the induction of Nrf2, BDNF, PGC-1, and Hsps, but also promotes neuroplasticity and neurogenesis .
11.4 Effect of Exercise on Oxidative Stress
Exercise is among the most promising strategies to decrease the risk of cognitive decline in old age (Middleton and Yaffe 2009) . Many studies have indicated that people who regularly exercise in mid- and late life have lower risk of developing cognitive impairment and dementia (Rovio et al. 2005). In addition seniors, who exercise have slower rates of cognitive decline than those who are inactive (Yaffe et al. 2001) supporting the view that exercise can improve cognition in elderly people. However, results are less consistent. Large, long-term, and double blind studies on human populations have not yet been performed (Angevaren et al. 2008). In addition, moderate physical exercise produces neuroprotective effects in age-related neurological disorders (Deslandes et al. 2009; Wichi et al. 2009). As stated in Chap. 10, neuroprotective effects of exercise can be explained on the basis of hormesis , which is defined as a bell-shaped (inverted U-shaped) dose response curve whereby moderate exercise stimulates resistance to stress and improves biological fitness, while too much exercise induces damage and inhibits neuronal function. This concept of hormesis can be extended to the effect of exercise on ROS and RNS production in the brain tissue (Radak et al. 2005, 2008; Um et al. 2008). At low levels ROS and RNS generated during moderate exercise serve as mediators for maintaining cellular milieu and transferring messages from one subcellular organelle to another to conduct physiological functions, such as neural cell proliferation and remodeling (adaptation), which is supported by increase in antioxidant and housekeeping enzyme activities (manganese superoxide dismutase and heme oxygenase), activation of transcription factor (Nrf2) and PPAR γ coactivator 1 (PGC-1), induction of heat shock proteins, DNA repair, and increased synthesis of brain-derived neurotrophic factor (BDNF) and Insulin-like growth factor-1(IGF-1) (Fig. 11.3). Thus, moderate exercise-mediated generation of ROS and RNS maintains physiological redox homeostasis. Exercise also upregulates the expression of anti-inflammatory cytokines , which inhibit inflammation and with time increase the immune system threshold for stress (Zaldivar et al. 2006) . These processes not only contribute to neuroprotection, neurogenesis , snd neuroplasticity , but also support maintenance of cognitive function and longevity. In contrast, intense and prolonged exercise not only increases levels of ROS and RNS, proinflammatory cytokines, catecholamine, adrenalin, and cortisol, but also stimulates lipid peroxidation (Steensberg et al. 2002; Navalta et al. 2010) and generation of superoxide anion through the xanthine oxidase activation, and increases the oxidized/reduced glutathione (GSSG/GSH) ratio (Sastre et al. 1992; Viña et al. 2000). Prolonged and exhaustive exercise causes oxidative stress, inflammatory response, and structural damage to muscle cells, as evidenced by an increase in the plasma activity of cytosolic enzymes, namely, lactic dehydrogenase and creatine kinase (Armstrong et al. 1983; Popovic et al. 2012). In intense and prolonged exercise also decreases astrocytic glycogen through the involvement of β-adrenergic receptor-mediated mechanism as shown by measuring the concentration differences in arterial–jugular venous glucose, lactate and oxygen, and their ratios (Larsen et al. 2008). Glycogen breaks down into lactate, as fuel for activated neurons, via increased noradrenaline (NA), histamine, 5-hydroxytryptamine (5-HT) and vasoactive intestinal peptide (Brown 2004). These processes may be associated with hypoglycemia, cognitive impairment, and central fatigue (Fig. 11.3). Collective evidence suggests that moderate regular exercise is a key component of a healthy lifestyle. It helps to prevent or delay the onset of visceral and neurological disorders. It is now becoming increasingly clear that above mentioned beneficial effects are lost when the exercise becomes exhaustive, indicating that the exercise intensity and duration are responsible for the beneficial or detrimental effects of exercise .
11.5 Effect of Exercise on Inflammation
A primary target of beneficial effects of exercise is the vasculature (Leung et al. 2008; Green 2009). Exercise increases vasodilation and improves vascular compliance (Green et al. 2011) which are likely a result of shear stress and cell stretch on both the endothelium and underlying smooth muscle (Green et al. 2011; Wang et al. 2007; Laughlin et al. 2008) . Exercise also protects the vasculature through a number of mechanisms (Katzmarzyk and Lear.2012) including reduction in inflammation (Mora et al. 2007). Collective evidence suggests that short-term exercise reduces the levels of TNF-α, IL-6, plasminogen activator inhibitor-1 (PAI-1) (Izadpanah et al. 2012), and cell adhesion molecules (Saetre et al. 2011), protects against media-intimal hyperplasia (Moreau et al. 2006; Pahkala et al. 2011) and smooth muscle cell hypertrophy, and strengthens the endothelial barrier (Ding et al. 2006). In addition, regular moderate exercise not only improves metabolic status and insulin sensitivity, but also induces anti-inflammatory effects (Stewart et al. 2007; Timmerman et al. 2008). During exercise, interleukin-6 (IL-6) not only stimulates expression of other anti-inflammatory cytokines such as interleukin-1 receptor antagonist (IL-1ra), interleukin-4 (IL-4), and interleukin-10 (IL-10), but also inhibits the production of the pro-inflammatory cytokine TNF-α (Stewart et al. 2007; Pedersen 2007; Timmerman et al. 2008). In addition, IL-6 increases fatty acid oxidation and insulin-stimulated glucose uptake and translocation of GLUT4 to the plasma membrane. Furthermore, IL-6 rapidly and markedly increases AMPK (AMP-activated protein kinase) and the metabolic effects of IL-6 were abrogated in AMPK dominant negative-infected cells. Furthermore, IL-6 also enhances lipid turnover through the stimulation of lipolysis. It is proposed that regular exercise induces suppression of TNF-α and thereby offers protection against TNF-α-mediated insulin resistance. Collective evidence suggests that IL-6, a multifactorial cytokine, regulates cellular and humoral responses in metabolic syndrome, a pathological condition, which is a risk factor for stroke , Alzheimer disease and depression (Farooqui et al. 2012; Farooqui 2013). In contrast, intense exercise produces increased cortisol levels (Van Bruggen et al. 2011), C-reactive protein (Mendham et al. 2011), and modest increases in other proinflammatory cytokines (Scott et al. 2011) .
11.6 Sleep and Induction of Oxidative Stress
Sleep is a behavioral state characterized by muscle atonia, activation of several brain areas, including the cortex, and phasically occurring eye movements, muscle twitches and changes in pulse rate, blood pressure and respiration (Cirelli and Tononi 2008). This type of sleep is called as REM sleep (rapid eye movement sleep). NREMS (non-REM sleep) is characterized by body rest. Sleep in human and animal depends on two major factors: a circadian regulator, defining the diurnal rhythm, and a homeostatic regulator defining the relationship between wake time and sleep time (Borbely 1982).
Sleep deprivation is a common feature in modern society. Sleep is absolutely essential for maintaining healthy physical, mental and emotional functions. Lack of good quality sleep affects physical, mental and emotional functions. Sleep deprivation not only affects learning and memory, but also results in the impairment of cognitive function (Potkin and Bunney 2012; Pace-Schott and Spencer 2011). Very little is known about signal transduction pathways associated with sleep deprivation. The mechanisms by which altered sleep deprivation affects health are unclear, but experimental studies suggest altered sleep may impact levels of cytokines, which are known to be important in regulating inflammation. Experimental sleep deprivation has been shown to acutely elevate proinflammatory cytokine levels including C-reactive protein (CRP) and interleukin-6 (IL-6) (Shearer et al. 2001; Haack et al. 2007; Patel et al. 2009) . However, it is not clear whether this pro-inflammatory effect observed with short-term sleep deprivation experiments persists chronically. Several studies have indicated that 24 h of REM sleep deprivation in mice disrupts the function of N-methyl-D-aspartate receptors (NMDA-R) in the dentate gyrus (Chen et al. 2006), and 72 h of REM deprivation in rats disrupted NMDA-R function in CA1 (McDermott et al. 2006). Importantly, application of glycine, which enhances NMDA-R function, reverses the effects of 24–48 h of sleep deprivation on LTP in area CA1 supporting the view that attenuation of NMDA-R function contributes to the hippocampal plasticity deficits observed after longer periods of sleep deprivation (McDermott et al. 2006). Recent studies have indicated that cAMP-PKA pathway is associated with LTP formation and memory consolidation (Abel et al. 1997; Havekes and Abel 2009). Downstream targets of the cAMP-PKA pathway associated with sleep deprivation include AMPA receptor (GluR1) or GluA1) serine 845 phosphorylation and cAMP response element binding protein (CREB), a transcription factor that plays a crucial role in memory and plasticity (Abel and Kandel 1998). Collective evidence suggests that disruption of sleep for a period shorter than 1 day has no effect on the basal rate of cell proliferation, but prolonged disruption of sleep causes marked alterations in signal transduction processes leading to a major decrease in hippocampal cell proliferation, cell survival and neurogenesis (Meerlo et al. 2009) .
Obstructive sleep apnea, a highly prevalent multifactorial sleep-related breathing disorder, is accompanied by snoring, disturbed sleep at night, and excessive daytime sleepiness. It is caused by repetitive and cyclic collapse of a narrow upper airway during sleep (Eckert and Malhotra 2008). Obstructive sleep apnea not only triggers sleep fragmentation, intrathoracic pressure swings and recurrent hypercapnia, but also induces a unique form of hypoxia called intermittent hypoxia. The molecular mechanisms underlying these risk factors are not clearly understood. However, repetitive episodes of intermittent hypoxia and reoxygenation not only produce high levels of ROS and RNS, but also activate hypoxia inducible factor (HIF-1α), nuclear factor kappa B (NF-κB), and tumor necrosis factor-α (TNF-α), interleukins 1 and 6 IL-1 and 6), vascular endothelial growth factor (VEGF), inducible nitric oxide synthase (iNOS), and caspase 3 (Carmeliet et al. 1998; Ryan et al. 2009). These processes initiate and promote oxidative and nitrosative stress along with inflammation. In addition, patients with obstructive sleep apnea also show increased levels of lipid peroxidation biomarkers and positive airway pressure (CPAP) therapy significantly decreases levels of these biomarkers (Lavie et al. 2004; Barcelo et al. 2000). Furthermore, treatment of rats exposed intermittent hypoxia with the superoxide dismutase (SOD) mimetic tempol restores vascular reactivity (Phillips et al. 2006). Similarly, rats exposed to chronic intermittent hypoxia also show increase in lipid peroxidation products, which is not only correlated with left ventricular dysfunction (Chen et al. 2005), but also with the development of endothelial dysfunction. Intravenous administration of the antioxidant vitamin C produces acute improvement in endothelium-dependent vasodilation (Grebe et al. 2006). These studies are supported by detected higher NF-κB and HIF-1 α activities in freshly harvested venous endothelial cells as well as higher levels of the oxidative stress marker nitrotyrosine in patients with obstructive sleep apnea (Jelic et al. 2008) and significant fall following CPAP therapy. Collective evidence suggests that sleep apnea induces oxidative and nitrosative stress along with the activation of NF-κB and HIF-1α, leading to stimulation of inflammatory signaling cascade, nitric oxide generation, angiogenesis, and apoptosis in the visceral tissues .
11.7 Sleep and Induction of Inflammation
It is well known that insufficient sleep and poor sleep quality are risk factors for inflammation-related conditions such as heart disease (Mullington et al. 2009) , immune dysfunction (Imeri and Opp 2009), and metabolic syndrome, a multifactorial condition, which is a risk factor for stroke , AD, and depression (Farooqui et al. 2012). As stated above, obstructive sleep apnea is accompanied by intermittent hypoxia. Repetitive short cycles of desaturation followed by rapid re-oxygenation of blood during the intermittent hypoxia play a pivotal role in the development of cardiovascular disease. It is also reported that the pathogenesis of sleep apnea also involves sympathetic nervous system overactivity, systemic inflammation and oxidative stress leading to endothelial dysfunction as well as metabolic dysfunction in the development of metabolic syndrome (Fig. 11.4) (McNicholas and Bonsignore et al. 2007; Ryan et al. 2009). Sleep disturbance is known to induce chronic oxidative stress and inflammation in visceral tissues as well as in the brain. Both oxidative stress and inflammatory processes in the brain impair learning and memory in rodents (Zhu et al. 2012). The molecular mechanisms associated with sleep disturbance are not fully understood. However, it is reported that sleep disturbance increases proinflammatory cytokine IL-6 levels and induce microglia activation in the mouse hippocampus, but not in the cortex (Zhu et al. 2012). In addition in brain, the expression of Homer1a is also increased after sleep loss, suggesting a role for sleep in the regulation of intracellular calcium homeostasis, particularly in protection and recovery from the calcium-pool changes induced by the prolonged neuronal activation imposed by extended wakefulness (Maret et al. 2007) . Accumulating evidence suggests that sleep disturbance-mediated neuroinflammation and oxidative stress in the mouse hippocampus impairs hippocampus-dependent learning and memory through alterations in LTP, which involve phosphorylation of cyclic AMP response element-binding protein (CREB) and the induction of genes such as Arc, brain-derived neurotrophic factor, and nerve growth factor-induced gene A, Homer, (Zhu et al. 2012; Ying et al. 2002; Silva 2003; Wallace et al. 1995). These observations support the view that there may be a functional relationship between neurogenesis , sleep, anxiety, memory and depression (Lucassen et al. 2010).
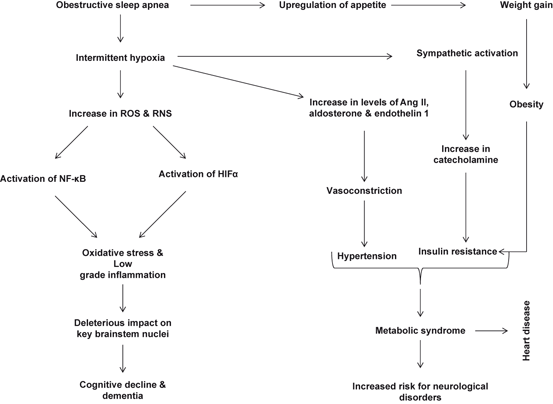
Fig. 11.4
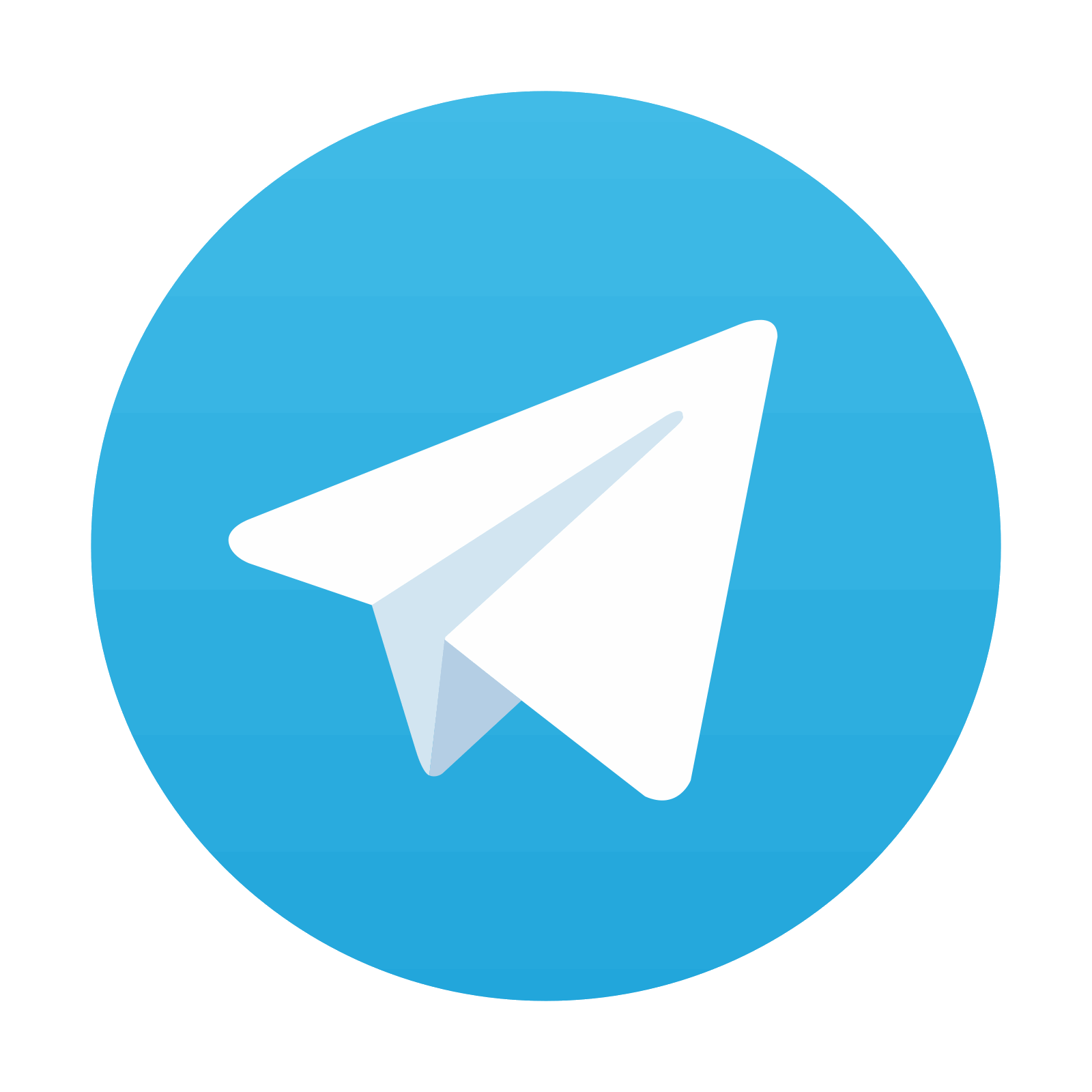
Effect of sleep apnea on neuroinflammation and oxidative stress. Reactive oxygen species (ROS), reactive nitrogen species (RNS), nuclear factor kappa-light-chain-enhancer of activated B cells (NF-κB), hypoxia-inducible factor 1-alpha (HIFα)
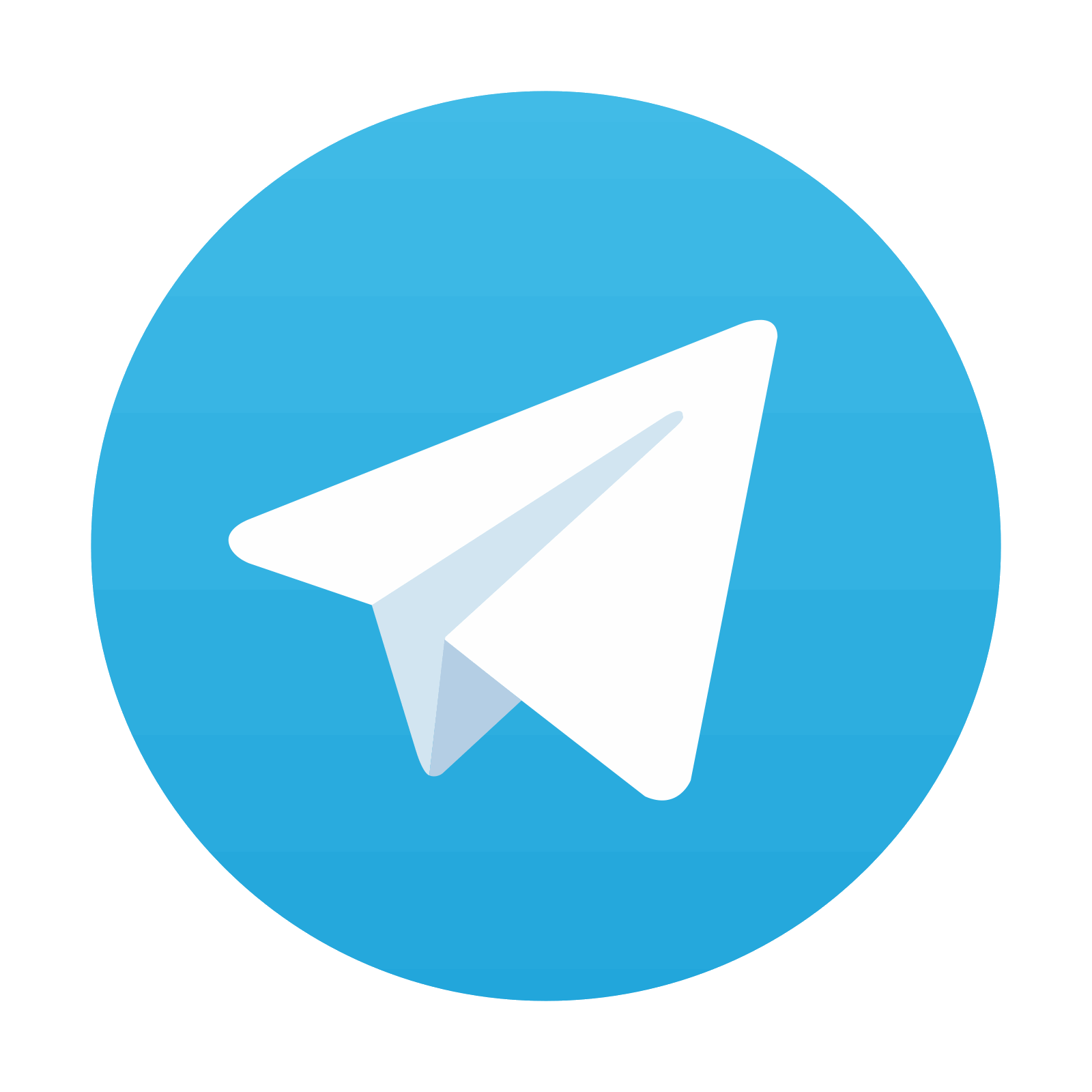
Stay updated, free articles. Join our Telegram channel
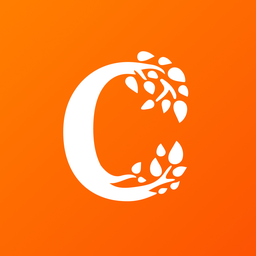
Full access? Get Clinical Tree
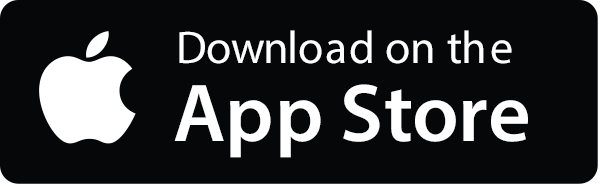
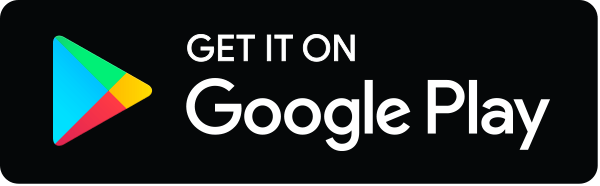
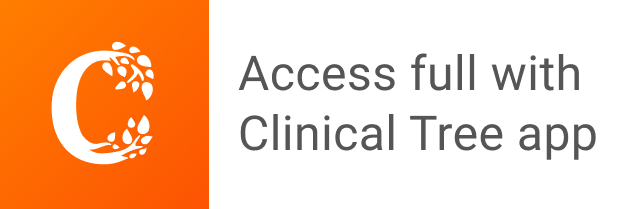